the Creative Commons Attribution 4.0 License.
the Creative Commons Attribution 4.0 License.
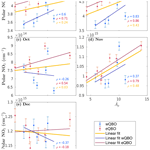
Evidence for energetic particle precipitation and quasi-biennial oscillation modulations of the Antarctic NO2 springtime stratospheric column from OMI observations
Emily M. Gordon
Annika Seppälä
Johanna Tamminen
Observations from the Ozone Monitoring Instrument (OMI) on the Aura satellite are used to study the effect of energetic particle precipitation (EPP, as proxied by the geomagnetic activity index, Ap) on the Antarctic stratospheric NO2 column in late winter–spring (August–December) during the period from 2005 to 2017. We show that the polar (60–90∘ S) stratospheric NO2 column is significantly correlated with EPP throughout the Antarctic spring, until the breakdown of the polar vortex in November. The strongest correlation takes place during years with the easterly phase of the quasi-biennial oscillation (QBO). The QBO modulation may be a combination of different effects: the QBO is known to influence the amount of the primary NOx source (N2O) via transport from the Equator to the polar region; and the QBO phase also affects polar temperatures, which may provide a link to the amount of denitrification occurring in the polar vortex. We find some support for the latter in an analysis of temperature and HNO3 observations from the Microwave Limb Sounder (MLS, on Aura). Our results suggest that once the background effect of the QBO is accounted for, the NOx produced by EPP significantly contributes to the stratospheric NO2 column at the time and altitudes when the ozone hole is present in the Antarctic stratosphere. Based on our findings, and the known role of NOx as a catalyst for ozone loss, we propose that as chlorine activation continues to decrease in the Antarctic stratosphere, the total EPP-NOx needs be accounted for in predictions of Antarctic ozone recovery.
- Article
(6544 KB) - Full-text XML
- BibTeX
- EndNote
In the polar stratosphere, the dominant source of odd nitrogen, NOx (NO+NO2), is produced via the oxidation of nitrous oxide, N2O (Brasseur and Solomon, 2005):
This reaction requires the presence of excited oxygen atoms O(1D), which are produced in the atmosphere by the photolysis of ozone (O3) and, thus, depend on the presence of sunlight. As a result, NO production via Reaction (1) only takes place outside of polar winter conditions. Following Reaction (1), the existing NO can be converted to NO2 by reaction with ozone:
As N2O production in situ in the polar stratosphere is insignificant, the polar stratospheric NOx production is highly dependant on the amount of N2O transported from the tropics (Brasseur and Solomon, 2005). This principal source of polar N2O is injected from the troposphere into the stratosphere at equatorial latitudes. It is then transported towards the polar regions by the large-scale Brewer–Dobson circulation. Recent work by Strahan et al. (2015) has shown that the phase of the QBO influences the transport of N2O from the “surf zone” to the polar vortex with a lag of 12 months. Further, their results (Fig. 1 of Strahan et al., 2015) indicate that the easterly phase of the QBO during June–July is also generally associated with positive N2O anomalies in the polar stratosphere between altitudes of ∼24 and 33 km in September, whereas the opposite is true for the westerly phase of the QBO. Notably for our study, these particular altitudes, at this time, are also affected by the large-scale transport of mesospheric air masses affected by energetic particle precipitation (Funke et al., 2014a).
The main pathway of NOx loss is via photolysis (Brasseur and Solomon, 2005). During polar night conditions when little to no sunlight is available, this results in a long chemical lifetime (weeks to months) for the NOx family. However, NOx can be removed from the lower stratosphere during the polar night via a process known as denitrification, which removes NOx when it is stored in the HNO3 reservoir. This requires the winter vortex to be cold enough that polar stratospheric clouds (PSCs) form. Denitrification occurs when reactive nitrogen (particularly NO2) is converted into HNO3 in the lower stratosphere (Santee et al., 1995). HNO3 is readily incorporated into PSCs, removing gaseous HNO3 from the lower stratosphere as it eventually falls into the troposphere via gravitational sedimentation (Brasseur and Solomon, 2005).
1.1 EPP indirect effect
It is now well established that precipitating energetic particles can drive large enhancements in NOx quantities in the polar atmosphere (see e.g. Seppälä et al., 2007a; Funke et al., 2014a, b). Energetic particle precipitation (EPP) is the flux of charged particles (protons and electrons) of solar and magnetospheric origin into the Earth's atmosphere. The charged particles are guided to the polar regions by the Earth's magnetic field. Once they reach the atmosphere, they ionize the main neutral gases (N2 and O2). The chain of ion-neutral reactions that follows the ionization then leads to increases in NOx species (this is known as “EPP-NOx”), particularly in the mesosphere and lower thermosphere (Brasseur and Solomon, 2005). EPP manifests as energetic electron precipitation (EEP) as well as proton precipitation, which in the form of solar proton events (SPEs) is the most extreme from of EPP (see e.g. Seppälä et al., 2014). SPEs are usually associated with coronal mass ejections (CMEs); thus, while the particles are highly energetic and have the ability to ionize as far down as the stratosphere, the events are short (hours to days) in duration and occur sporadically. Conversely, EEP is always present in some form and is mostly dependant on the solar wind speed (Funke et al., 2014b). Due to the lower energies of the electrons, EEP-driven in situ NOx increases typically occur in the mesosphere and above (Turunen et al., 2009). When EPP occurs over the winter pole, the mesospheric NOx has a long chemical lifetime and can be transported downward into the stratosphere inside the polar vortex. Once in the stratosphere, these NOx enhancements are effective at catalytically destroying ozone (see e.g., Jackman et al., 2008, and references therein). As NOx is not formed from N2O during winter, EPP becomes a significant contributor to the polar winter NOx budget (Funke et al., 2014b).
1.2 Previous work
Several previous studies have examined the effects of EPP on polar winter NOx or the wider NOy family, the latter of which includes both NOx and its reservoir species such as NO3, N2O5, HNO3, and ClONO2. We will summarize the findings of the key observational works, with particular focus on those with Southern Hemisphere (SH) or NOx transport aspects, in the following. Note that we will use the term NOx or NOy depending on which the study in question addressed.
Randall et al. (1998) reported stratospheric NO2 observations from the Polar Ozone and Aerosol Measurement (POAM II) instrument over three polar late winter–early spring periods. They found evidence to suggest that NOx from the SH polar mesosphere was transported down into the stratosphere inside the polar vortex during the winter. They also suggested that the observed enhanced levels of stratospheric NOx in 1994 could have been, at least partially, due to production by EPP that took place at higher altitudes before the downward transport. Based on their analysis, Randall et al. (1998) suggested that NOx transported to stratosphere from the mesosphere and above during the polar winter should be observable in Antarctic NO2 column measurements.
Siskind et al. (2000) used Halogen Occultation Experiment (HALOE) observations from the Upper Atmosphere Research Satellite (UARS) satellite between 1991 and 1996 to track NOx enhancements in October in the SH polar region. They found that the year-to-year variability in NOx inside the polar vortex followed variability in the wintertime mean auroral geomagnetic activity index, the Ap index, which is a measure now frequently used for overall EPP levels (Matthes et al., 2017). At the time, they found that the peak NOx enhancements from “auroral” activity corresponded to around 3 %–5 % of the total NOx generated from N2O. Studies of NOx enhancements following the large Halloween SPEs in October–November 2003 also revealed large quantities of NOx in the Northern Hemisphere (NH) in January (Jackman et al., 2005). However, dynamical effects, driven by the following major sudden stratospheric warming (SSW), indicated that this NOx was unlikely to have originated from the SPEs (Seppälä et al., 2007b). The NOx increases were more likely a result of the large amount of EEP that was present during the polar winter combined with downward descent. For example, Randall et al. (2005) used observations from a number of satellite instruments to show that the springtime NOx increases in the NH were influenced by strong downward descent in January 2004, which brought an excess amount of NOx down to the stratosphere. Randall et al. (2007) used Atmospheric Chemistry Experiment Fourier transform spectrometer (ACE-FTS) and HALOE observations to show that the peak upper stratospheric EPP-NOx was highly correlated with EEP levels from 1992 to 2005, which continued until September in the SH. Dynamics influencing the polar vortex is one of the main reasons that relations between NOx observations and EPP break down. This is particularly important in the NH, where the polar vortex is more susceptible to SSWs. Randall et al. (2007) also found that the largest EPP-NOx enhancements occurred during the declining phase of the solar cycle, when more high-speed solar wind streams, driving EEP, are likely to occur.
Using tracer correlations to quantify EPP-NOx vs. NOx from N2O oxidation, Randall et al. (2007) suggested that the maximum EPP-NOx enhancements comprised up to 40% of the total polar NOy (total reactive nitrogen, ) budget. Seppälä et al. (2007a) contrasted the average wintertime Ap levels with the polar winter upper stratospheric–lower mesospheric NOx observations from the Global Ozone Monitoring by Occultation of Stars (GOMOS) instrument onboard the Envisat satellite, finding a nearly linear relationship between the two from 2002 to 2006 for both hemispheres. Funke et al. (2014b) used Michelson Interferometer for Passive Atmospheric Sounding (MIPAS) observations, also from Envisat, of NOy to quantify the amount of EPP-NOy in the polar winter. Analogous to Randall et al. (2007), they found that EPP-NOy accounted for up to 40% of the wintertime polar NOy. Funke et al. (2014a) then correlated Ap and EPP-NOy in the wintertime and concluded that the strong relationship between Ap and EPP-NOy supports using Ap as a proxy for tracking EPP-NOy production in the SH wintertime.
1.3 This work
Here, we use stratospheric NO2 column observations from the Ozone Monitoring Instrument (OMI) onboard the Aura satellite to investigate EPP as a source of NOx variability in the Antarctic late winter–spring. We have a relatively long satellite period (2005–2017) in which to analyse how this NOx propagates in the following springtime, and whether this is detectable in the NO2 column. We also analyse how the phase of the QBO affects the contribution of EPP-NO2 to the total NO2 column in springtime. The QBO influence could likely be due to a combination of two effects: (1) the QBO phase influences the transport of N2O to the polar region (Strahan et al., 2015), resulting in an increased background NOx source at key times and key altitudes during easterly QBO years (opposite for westerly QBO); and (2) QBO conditions influence polar temperatures, which may affect the probability of PSC formation. As PSC formation is linked to denitrification, there may be a connection between the removal of NOx and the QBO. To test for the latter, we will provide a simple analysis of HNO3 and temperature observations from the Microwave Limb Sounder (MLS), also onboard Aura (a more detailed analysis of PSC observations would be required to test this further).
2.1 OMI NO2 observations
We use stratospheric NO2 column observations from the Dutch–Finnish-built Ozone Monitoring Instrument (OMI) onboard NASA's Aura satellite from August to December between 2005 and 2017 (v3, Level 2 daily gridded NO2; see Krotov et al., 2019 and Krotkov et al., 2017). The daily gridded data have a horizontal resolution. In our analysis, we use data from latitudes poleward of 50∘ S. Note that all latitudes are geographic latitudes in the following. Aura is in a Sun-synchronous orbit in the “A-train” constellation (orbital altitude of 705 km, inclination of 98∘, and 16 d repeat cycle), with the ascending node crossing the Equator at approximately 13:45 LST daily (Schoeberl et al., 2008). As a result, OMI measurements take place at the same locations each year. While NO2 has a notable diurnal cycle, using observations from the same sunlit locations (thus the same local times) each year minimizes this effect in our analysis.
The OMI NO2 data are provided as total column as well as separated tropospheric and stratospheric columns. This separation is based on the location of the tropopause. Here, we only use the OMI stratospheric column observations. The effective vertical range of the stratospheric column based on the OMI averaging kernels corresponds to ∼15–35 km. At these altitudes, NO2 makes up about 80 % of the total NOx during daytime (see Brasseur and Solomon, 2005, chap. 5.5). As this corresponds to a large fraction of the total NOx, we take the OMI NO2 column measurements to represent a reasonable proxy for the variation in total NOx. The algorithm for the OMI column separation is described in Bucsela et al. (2013). OMI measures backscattered solar radiation from the atmosphere. Thus, observations are only available for solar illuminated locations – there is no coverage during polar night conditions. The latitudinal coverage is illustrated in Fig. 1, which presents the zonally averaged mean NO2 column for the period under investigation (2005–2017). The figure shows how the NO2 column varies in the polar springtime, with increasing amounts of NO2 in the stratosphere as time progresses due to its release from its reservoirs (Dirksen et al., 2011). The error in the individual NO2 column measurement is estimated to be less than 2×1014 molecules cm−2; however, in areas with low levels of tropospheric pollution (such as the southern polar region), this error is considerably less (Bucsela et al., 2013). Since June 2007, OMI NO2 has experienced an issue known as the row anomaly (RA), which affects certain fields of view. All RA-affected measurements have been excluded here, leaving around 2×105 observations poleward of 60∘ S per day for the analysis period.
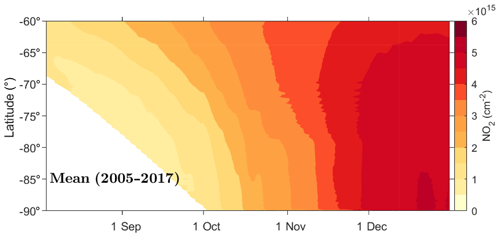
Figure 1The OMI 3 d running mean zonally averaged NO2 column for the time period from 2005 to 2017. The contour interval is 0.5×1015 cm−2. The white area at high latitudes in August–September indicates polar night conditions, during which OMI observations are not available.
The August–December monthly mean polar (60 to 90∘ S) average zonal mean NO2 columns for each year are listed in Table 1.
2.2 MLS observations
We use HNO3 and temperature profiles from NASA's Microwave Limb Sounder (MLS), which is also onboard the Aura satellite (Manney et al., 2015; Schwartz and Read, 2015). This study uses the version 4.2 product with data screened according to Livesey et al. (2017). The latitude range used here is 60 to around 82∘ S, and the pressure range used is approximately 100 to 10 hPa. MLS HNO3 profiles have been validated by Santee et al. (2007), using data from both the HNO3 240 GHz radiometer (for pressures ≥22 hPa) and the HNO3 190 GHz radiometer (for pressures ≤15 hPa). MLS HNO3 has a vertical resolution of 3–4 km in the lower–middle stratosphere (used here), and the precision of individual profiles is around 0.6 ppbv in this region. The estimated error in these profiles is no more than 10 %. MLS temperature profiles have been validated by Schwartz et al. (2008) with a precision of around ±0.6 K on individual profiles between 100 and 10 hPa.
2.3 EPP proxy
The geomagnetic activity index, Ap, is a well-established proxy for EPP (see e.g. Matthes et al., 2017; Funke et al., 2014a), and it is used here to estimate the overall levels of EPP for each polar winter under investigation. From 2005 to 2017, the mean of winter Ap was 8.3, reflecting the relatively low overall solar activity during solar cycle 24 (the solar cycle 23 average was 12.9). To estimate the overall EPP activity during each winter, we calculate the mean Ap for the May–August period of each year. These means are referred to as hereafter and are provided in Table 1. We designate high (H-) winters as those with , i.e. a value higher than the average for 2005–2017. Similarly, we designate low winters (L-) as those with . The variation in winter throughout this study is shown in Fig. 2. This figure captures the 11-year solar cycle fairly well, with a minimum around 2009 and a maximum around 2015.
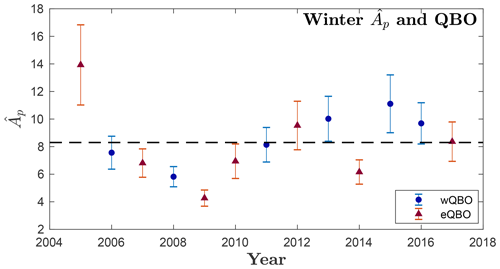
Figure 2Mean wintertime Ap () for each year of this study with the error bars indicating 2 times the standard error of the mean (±2SEM). The dashed line indicates the average for this study (8.3). The direction of the QBO at 25 hPa in May is indicated: red triangles represent the eQBO, and blue circles represent the wQBO.
Several previous works have used tracer correlations to extract only the NOx produced by EPP (or NOy when information on the NOx reservoirs is available; see e.g. Randall et al., 2007 and Funke et al., 2014b). When tracer information is not available, other works have investigated the variability in odd nitrogen resulting in variability in EPP levels (usually proxied by Ap; see e.g. Seppälä et al., 2007a). Here, we focus on finding evidence of the EPP contribution to column observations. As we do not have mesosphere–stratosphere descent tracer observations available from OMI, we are unable to use the tracer correlation methods. To overcome this, we perform correlation analysis for both latitudinal coverage and polar average NO2 observations to find evidence of Ap-driven variability in the Antarctic NO2 column. All correlations between the NO2 columns and are based on the Spearman rank correlation (Spearman ρ), as it more robustly accounts for any non-linear relationships (Wilks, 2011) while still interpreting linear trends where present. Statistical significance is defined here as correlations significant at the ≥95 % level (i.e. p ≤0.05).
2.4 Quasi-biennial oscillation
To investigate the potential QBO effect in the Antarctic atmosphere, we estimate the phase of the QBO from the 25 hPa level zonal mean zonal wind (Naujokat, 1986) near the Equator in May each year. For use of the 25 hPa level in the Southern Hemisphere, see Baldwin and Dunkerton (1998). We designate years when the zonal mean zonal wind direction is easterly as the easterly QBO (eQBO) and westerly as the westerly QBO (wQBO). The QBO direction for each year of the study is indicated in both Table 1 and Fig. 2. Figure 2 illustrates the approximately biennial nature of the oscillation, with the direction changing almost every year.
3.1 NO2 anomalies
We first investigate the anomaly from the mean for each of the four different categories of this study: eQBO H-, eQBO L-, wQBO H-, and wQBO L-. This is to show how the NO2 column evolves in the springtime under the different conditions and to further justify the splitting of years based on the QBO phase. Figure 3 presents the average anomaly, (i.e. the mean, as shown in Fig. 1, is deducted) for each of the four different categories of this study. We can see that the winter affects the column NO2 present in the spring: years with H- (Fig. 3a, c) have more positive anomalies from August to November, especially at the highest latitudes. In Fig. b and c, the month of October is highly variable, with both panels showing regions of positive and negative anomaly. For low years (Fig. 3b, d), early spring is not consistently positive or negative; however, November displays negative anomalies at high latitudes. The combined influence of the QBO and appears to be most significant for H- eQBO years and L- wQBO years (Fig. 3a, d). These show consistent but opposite behaviour throughout the spring, with H- eQBO years being the most favourable for NO2 and wQBO L- being the least favourable.
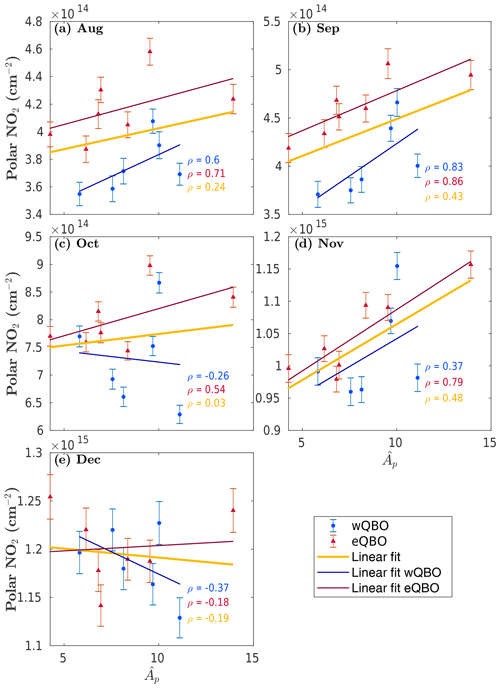
Figure 4 vs. the monthly average cos (latitude) area-weighed NO2 column density averaged over the region from 60 to 90∘ S (where available, see text) from August to December (months are shown as individual panels). Red triangles indicate years with eQBO, and blue circles indicate years with wQBO. The yellow line shows a least squares linear fit to all data, the red line shows the eQBO years only, and the blue line shows the wQBO years only. The Spearman ρ (correlation coefficient) for each set is shown in each panel using the corresponding colour, e.g. red ρ corresponds to the correlation coefficient for eQBO years. Error bars are ±2SEM.
3.2 Mean SH polar columns
Figure 4 presents the and the mean polar (60–90∘ S) NO2 column for each year (2005–2017) and for each individual month from August to December. This is to investigate whether a relationship exists between size and the NO2 column, and how this is affected by the QBO phase. The mean polar columns are area weighted by the cosine of latitude. The phase of the QBO in the preceding May is indicated: red triangles correspond to eQBO conditions, and blue circles correspond to wQBO conditions. Least squares linear fits for all years, wQBO years, and eQBO years are included in each panel to guide the eye. The Spearman correlation coefficient (ρ) for each month for all years (yellow) and for eQBO (red) and wQBO (blue) years only are also included in the panels. Note that as the OMI measurement field gradually increases from an initial maximum latitude of around 68∘ S in August to 90∘ S by the end of September, the total NO2 column values do not initially fully encompass the entire polar region (60–90∘ S). The missing data in August and September are treated as missing values in the mean calculation and, as such, do not contribute to the mean in these figures.
The results shown in Fig. 4 suggests that a correlation between and the stratospheric NO2 column occurs in August, September, and November. This is consistent with Fig. 3. Furthermore, there is a clear positive correlation for eQBO years from August to November, whereas the positive correlation in August and September disappears in October for wQBO years. While the wQBO November linear fit is close to the total fit, the individual years show large variability. In general, wQBO years have consistently lower column NO2 values, especially in August and September. The generally reduced levels of NO2 during wQBO conditions is compatible with the analysis of Strahan et al. (2015), which indicated that the altitudes where Funke et al. (2014a) reported EPP-NOy enhancements in later winter–early spring have consistently lower (higher) levels of the dominant NOx source, N2O, during wQBO (eQBO).
To contrast our results with previous extensive work by Funke et al. (2014a), we repeated the analysis presented in Fig. 4 using gigamoles (Gmol, see Funke et al., 2016) for the monthly mean polar NO2 columns. This figure is included in the Appendix as Fig. A1 and shows the least squares linear fits, with the corresponding parameters given in each panel. This allows us to estimate the EPP contribution to the lower stratosphere (∼15–35 km) NO2 in the spring, analogous to Funke et al. (2014a). For example, in September (Fig. A1b in the Appendix), the approximate contribution from EPP in eQBO years to the polar stratospheric NO2 column is +0.023 Gmol/. The largest contribution to OMI lower stratospheric NO2 from EPP occurs in November, with the corresponding values of +0.073 (eQBO), +0.072 (wQBO), and +0.066 (all years) Gmol/ respectively. Funke et al. (2014a) (see their Fig. 10, showing excess EPP-NOy in the stratosphere–lower mesosphere in early spring) found an increase in SH polar EPP-NOy of around +0.0698 Gmol/Ap in September. Contrasting this with our results of +0.066 Gmol/ in November, it seems that a large fraction of the EPP-NOy detected by Funke et al. (2014a) is maintained in the polar region and is able to reach the lower stratosphere, where it can still be detected as NO2 in November. Note that Funke et al. (2014a) use a weighted Ap scheme.
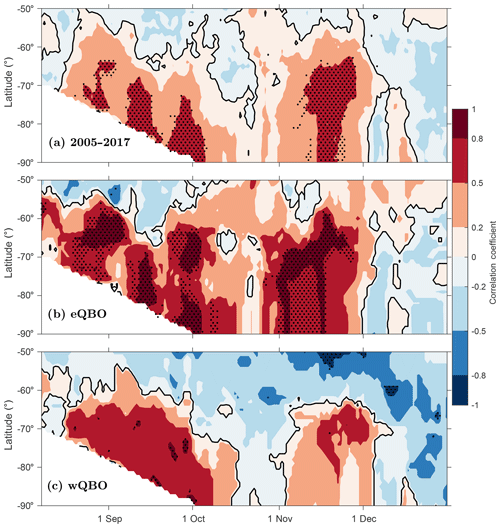
Figure 5(a) Correlation of and the 7 d running mean NO2 column density for August–December for all years; (b) correlation of and the 7 d running mean NO2 column density for years with eQBO only; (c) correlation of and the 7 d running mean NO2 column density for years with wQBO only. All figures have a 1∘ latitudinal resolution. Contour levels are shown for [−1, −0.8, −0.5, −0.2, 0, 0.2, 0.5, 0.8, 1], and the zero contour is indicated using black. Stippling shows regions with a correlation significant at the ≥95 % level.
3.3 Latitudinal correlations
Figure 5 shows the latitudinal extent of the correlation between and the 7 d running mean NO2 column for latitudes from 50 to 90∘ S averaged over 1∘ latitude bins. Stippling indicates that the correlation is significant at the ≥95 % level. This shows how the correlation between and the NO2 column evolves over time and latitude as well as different QBO phases. Figure 5a presents the correlation when all years are taken into account. A significant positive correlation occurs in late August and varies throughout September; it is then significant again in November. October and December show little to no significant correlation. Figure 5b shows the correlation for eQBO years only. There are areas of statistically significant positive correlations in all months except December. In October, significant correlations only occur at the very beginning of the month. High positive correlations are still present from 60 to 90∘ S from early to mid-November, providing the first evidence that the indirect EPP-NOx effect lasts well into the SH spring. Figure 5c presents the correlation for years with wQBO only. While positive correlations are present throughout August and September, only small regions are found to be statistically significant. October marks a shift towards a negative correlation (not statistically significant) at all latitudes. In November, the correlations turn positive once more, but these are once again not statistically significant.
The results shown in Fig. 5 suggest that the NO2 increases at high polar latitudes in September are due to increased EPP/geomagnetic activity, as strong correlations between NO2 and occur in all panels. They also imply that increases in NO2 in November can be due to a combination of high EPP activity and eQBO, whereas wQBO appears to reduce the occurrence of any EPP-induced NO2 increases.
3.4 The polar vortex influence in October
The correlations presented in the previous sections were found to have fewer occurrences of statistical significance in October than the surrounding months. As this time of year marks the typical break-up period of the polar vortex (Hurwitz et al., 2010) and knowing that the descent of EPP-produced NOx is limited to inside the polar vortex (as previously demonstrated for October by Siskind et al., 2000), we will now investigate October separately, taking the polar vortex into account.
To account for effects from potential asymmetries in the shape of the polar vortex in October in our zonal mean calculations, we repeated the earlier analysis for measurements located inside the vortex. To establish the location of the edge of the polar vortex, we utilized the OMI co-located ozone column measurements (Bhartia, 2012): ozone-depleted air is isolated within the vortex until the vortex break-up, typically in late November (Kuttippurath and Nair, 2017). Based on this, we assume that measurement locations poleward of 50∘ S with a corresponding stratospheric ozone column of <245 DU are inside the polar vortex. We perform this assessment for every day of October during the study period in order to find the daily vortex extent. This is then used to locate NO2 that is inside the vortex for every day in October during the study period. An example of how the ozone column and the estimated vortex edge are reflected in the NO2 column measurement for 1 day of the study (19 October 2014) is shown in Fig. 6. Note that we are unable to use this method on months prior to October due to OMI's viewing method and the ozone column not being low enough to detect a clear vortex edge.
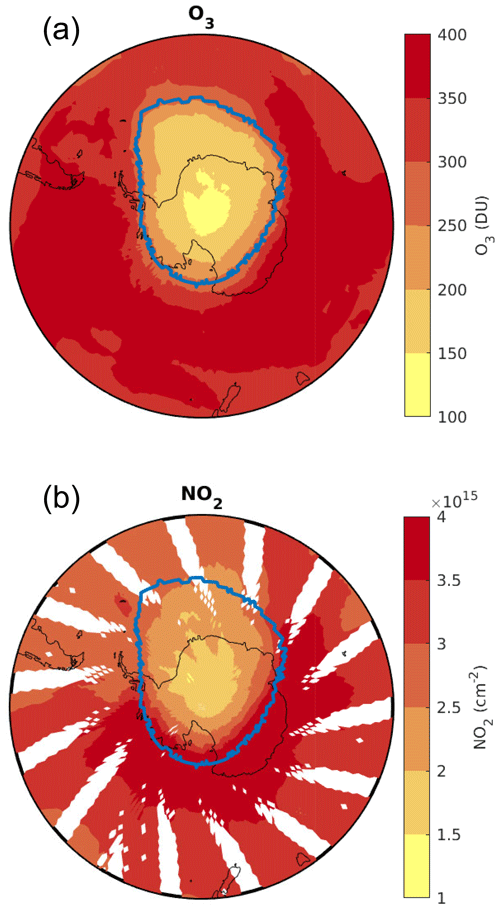
Figure 6Vortex edge identification based on the OMI ozone column for 19 October 2014. Panel (a) shows the OMI measured ozone column density with the 245 DU contour highlighted. Panel (b) shows the NO2 stratospheric column with the 245 DU ozone contour highlighted. This method is repeated for each individual day in October throughout the study period.
Figure 7a shows the October results (as in Fig. 4c) when only observations inside the polar vortex are included. For eQBO, we find that the observations are now much closer to the linear fit than in Fig. 4c, implying that the earlier disappearance of correlation was likely due to variations in the shape of the polar vortex in October.
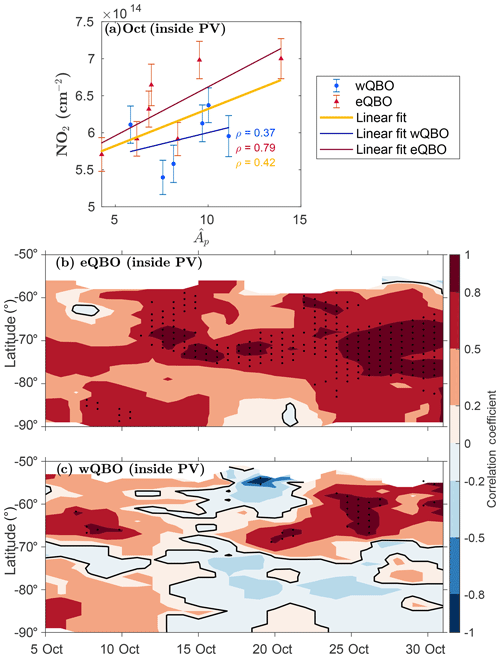
Figure 7(a) vs. the average, cos (latitude) area-weighted NO2 for the region from 60 to 90∘ S for observations inside the polar vortex (PV). Red triangles correspond to eQBO years, and blue circles correspond to wQBO years. The yellow line represents a linear fit to all data points, the red line represents a linear fit for eQBO years only, and the blue line represents a linear fit for wQBO years only. ρ values are displayed as in Fig. 4. Error bars indicate the 95 % confidence interval for the mean. (b) Correlation of with the 5 d running mean NO2 column inside the polar vortex for eQBO years. Contour levels are shown for [−1, −0.8, −0.5, −0.2, 0, 0.2, 0.5, 0.8, 1] with an additional black line for the zero contour. The stippling indicates that correlations are significant at the ≥95 % level. Panel (c) is the same as panel (b) but for wQBO years.
Similarly, for the horizontal distribution of the correlations (Fig. 7b), we now find high correlations for eQBO years throughout October. This again implies that the lack of a correlation in October is due to the distorted shape of the polar vortex being smeared out by the calculation of zonal means, and the effect of EPP on the NO2 column is significant through October. The reappearance of correlations in eQBO years in November in Fig. 5b is likely a mixing effect, with the breakdown of the polar vortex around this time leading to vortex air being mixed with extra-vortex air which results in the NO2 distribution not being as skewed as it was when contained in the vortex. Figure 7c also shows higher correlation with more instances of significance in wQBO years in October than in Fig. 5c, although this is more variable than in eQBO years (which is consistent with Fig. 5, where wQBO years show lower correlation). Although the Ap index has generally been lower in the past decade than it was during the 1991–1996 period investigated by Siskind et al. (2000), considering observations only in the vortex still shows the same, strong linear relationship found in that study.
4.1 Influence of the QBO
As shown in Figs. 3, 4, and 5a, our results suggest that the phase of the QBO influences the SH polar EPP-NOx signal in the spring months. The results of Strahan et al. (2015) suggest that the eQBO phase in early winter leads to increased N2O between altitudes of ∼24 and 33 km in September: Fig. 1 of Strahan et al. (2015) shows a positive anomaly for N2O in September in eQBO years (and the opposite for wQBO). Although their designation of the QBO phase differs slightly from ours, this would not largely affect their comparability, as our designations only differ for 1 year of a 10 year study. Our results suggest that although there is an increased pool of N2O in the polar region during eQBO (contributing to a larger NO2 column from N2O oxidation), EPP still contributes a clear fraction to the overall SH polar stratospheric column NO2 in the springtime. Taking the QBO phase into account, this EPP contribution during spring is generally more pronounced. We note that the high correlation with is only present until September for wQBO years, whereas it lasts until November for eQBO years. It is not clear why this is the case for wQBO. A further analysis of possible reasons behind this discrepancy is beyond the scope of this work.
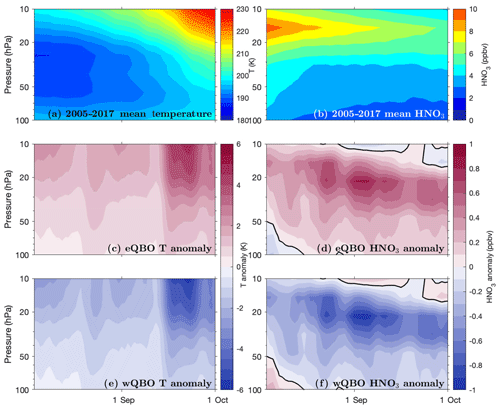
Figure 8(a) The 3 d running mean MLS temperature from 2005 to 2017 (5 K contour interval), and (b) the 3 d running mean MLS HNO3 from 2005 to 2017 (1 ppbv contour interval). Panels (a) and (b) are both averaged over the region from 60 to 82∘ S for the lower stratosphere for the late winter–early spring period from 2005 to 2017. (c) The HNO3 anomaly (the mean shown in panel a has been subtracted) for years with eQBO (the contour interval is 0.1 ppbv, and the black contour shows the zero anomaly). (d) The temperature anomaly (mean shown in panel b has been subtracted) for years with eQBO (the contour interval is 1 K, and the black contour shows the zero anomaly). Panel (e) is the same as panel (c) but for years with wQBO, and panel (f) is the same as panel (d) but for years with wQBO.
4.2 Possible influence of PSCs and denitrification
Here we discuss possible reasons for the consistently lower amounts of NO2 in wQBO years found in Fig. 4.
Baldwin and Dunkerton (1998) found that the polar vortex is colder during winters with wQBO. A colder polar vortex results in a higher likelihood of polar stratospheric cloud (PSC) formation (Brasseur and Solomon, 2005). As discussed in Sect. 1, PSCs affect the heterogeneous chemistry in the polar region, leading to the denitrification of the lower stratosphere (Dirksen et al., 2011). One of the possible reasons for the lower NO2 column during wQBO found here could be enhanced denitrification as a result of lower temperatures in the vortex that bring about enhanced PSC formation. In the following, we will perform a simple analysis to test for signs of this from existing MLS observations, although a more detailed analysis of PSC observations would be required for robust conclusions.
To test whether the QBO phase affects denitrification in the Antarctic stratosphere, we analysed temperature and HNO3 observations from the MLS (see Sect. 2.2). Figure 8a and b show the mean temperature and HNO3 respectively, each averaged over the region from 60 to 82∘ S for 2005–2017 over the late winter–early spring period, i.e. when the polar vortex is coldest and PSCs are forming. Figure 8c and e show the anomalies from the mean temperature for eQBO and wQBO years respectively. Figure 8d and f present the anomaly from the mean HNO3 mixing ratio for eQBO and wQBO years respectively. The vertical pressure range of all of the panels is 100 to 10 hPa which corresponds to an altitude range of approximately 17 to 32 km. Figure 8 suggests that eQBO years tend to have more HNO3 (up to 1 ppbv) and a higher temperature (up to 4 K) throughout this period, whereas wQBO years show a consistently negative anomaly in HNO3 (down to −1 ppbv) and a lower temperature (down to −4 K). Colder temperatures would likely lead to more PSC formation and, thus, more HNO3 being removed from the stratosphere (more denitrification) in wQBO years (than in eQBO years). It should, however, be noted here that the link between PSC coverage and QBO modulation of polar temperature via the Holton–Tan mechanism is still under debate (see e.g. Strahan et al., 2015).
This study provides new evidence of the EPP contribution to the Antarctic stratospheric NOx column in the late springtime using OMI (Aura) stratospheric NO2 observations. This is one of the few studies to use stratospheric NO2 data from OMI and highlights the value of long time series of stratospheric NO2 from nadir-viewing instruments. Our analysis shows that the influence of the QBO is able to mask the stratospheric EPP-NOx signal in satellite observations in a way that, to our knowledge, has not previously been accounted for: considering the phase of the QBO makes the contribution from EPP more pronounced in the NO2 column, and signals of enhanced EPP-NOx in the polar stratospheric column can be detected until late November.
Previously, Funke et al. (2014a) analysed EPP-NOy observations, and they were able to attribute SH enhancements with an average Ap dependence of +0.0698 Gmol/Ap to early spring months (September). Here, we show that this reactive nitrogen lingers, entering the lower stratosphere in the form of NO2 at an average Ap dependence of +0.066 Gmol/ in November.
We present evidence of the contribution from EPP-NOx in the Antarctic stratosphere at a time when halogen-activated ozone loss is taking place. NOx is well known to react with both ozone and active halogens, catalytically destroying the former and driving the latter to its reservoirs (Brasseur and Solomon, 2005). Antarctic ozone loss has been found to be reduced in years with eQBO (Garcia and Solomon, 1987) due (at least in part) to the increased vortex temperatures hampering chlorine activation on PSCs (Lait et al., 1989). Our results suggest that as chlorine activation continues to decrease in the Antarctic stratosphere following the Montreal Protocol (Solomon et al., 2016), the total EPP-NOx (in addition to SPEs as pointed out by Stone et al., 2018) should be accounted for in predictions of Antarctic springtime ozone recovery. Future studies should investigate the role of the larger EPP-NOx fraction when investigating the net effect of NO2 on the fragile ozone chemistry in the springtime.
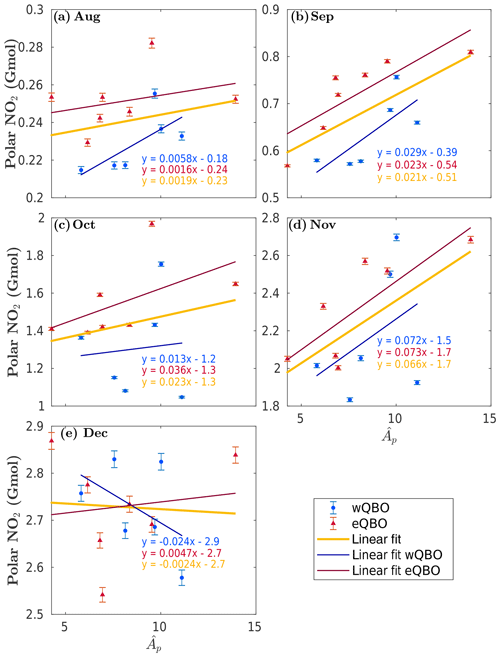
Figure A1The same as Fig. 4 but with the monthly average polar NO2 expressed in gigamoles (Gmol). Each panel shows the linear least squares fit to data points (colour coding is the same as that in previous figures), including the fit equations (y= NO2, ). Red triangles are years with eQBO, and blue circles are years with wQBO. The yellow linear fit is a best-fit line for all of the data in each plot, the red line fits only eQBO data, and the blue line fits only wQBO data.
All data used here are open access. Ap data can be accessed at http://wdc.kugi.kyoto-u.ac.jp/kp (last access: 22 January 2019) (World Data Center for Geomagnetism, 2019); QBO data can be accessed at https://www.geo.fu-berlin.de/en/met/ag/strat/produkte/qbo (last access: 27 December 2019) (for more information please contact Markus Kunze at markus.kunze@met.fu-berlin.de); and OMI (https://doi.org/10.5067/Aura/OMI/DATA2018; Krotov et al., 2019) and MLS (https://disc.gsfc.nasa.gov/datacollection/ML2HNO3_003.html, last access: 29 August 2019; EOS MLS Science Team, 2011a) data can be accessed at https://disc.gsfc.nasa.gov/datacollection/ML2T_003.html (last access: 29 August 2019) (EOS MLS Science Team, 2011b).
EG and AS planned the study. EG carried out the analysis with support from AS. JT provided expertise on OMI observations. EG and AS lead the writing of the paper with comments from all authors.
The authors declare that they have no conflict of interest.
We are grateful to the World Data Center for Geomagnetism, the Freie Universität Berlin, and the National Aeronautics and Space Administration for providing open access to the data sets used in this study. We would like to thank Dan Smale and Cora Randall for their useful discussions and valuable feedback.
This paper was edited by Thomas von Clarmann and reviewed by two anonymous referees.
Baldwin, M. P. and Dunkerton, T. J.: Quasi‐biennial modulation of the southern hemisphere stratospheric polar vortex, Geophys. Res. Lett., 25, 3343–3346, https://doi.org/10.1029/98GL02445, 1998. a, b
Bhartia, P. K.: OMI/Aura Ozone (O3) Total Column Daily L2 Global Gridded 0.25 degree × 0.25 degree V3, https://doi.org/10.5067/Aura/OMI/DATA2025, 2012. a
Brasseur, G. P. and Solomon, S.: Aeronomy of the Middle Atmosphere, Springer, 2005. a, b, c, d, e, f, g, h
Bucsela, E. J., Krotkov, N. A., Celarier, E. A., Lamsal, L. N., Swartz, W. H., Bhartia, P. K., Boersma, K. F., Veefkind, J. P., Gleason, J. F., and Pickering, K. E.: A new stratospheric and tropospheric NO2 retrieval algorithm for nadir-viewing satellite instruments: applications to OMI, Atmos. Meas. Tech., 6, 2607–2626, https://doi.org/10.5194/amt-6-2607-2013, 2013. a, b
Dirksen, R. J., Boersma, K. F., Eskes, H. J., Ionov, D. V., Bucsela, E. J., Levelt, P. F., and Kelder, H. M.: Evaluation of stratospheric NO2 retrieved from the Ozone Monitoring Instrument: Intercomparison, diurnal cycle, and trending, J. Geophys. Res.-Atmos., 116, D08305, https://doi.org/10.1029/2010JD014943, 2011. a, b
EOS MLS Science Team: MLS/Aura Level 2 Nitric Acid (HNO3) Mixing Ratio V003, Goddard Earth Sciences Data and Information Services Center (GES DISC), Greenbelt, MD, USA, available at: https://disc.gsfc.nasa.gov/datacollection/ML2HNO3_003.html (last access: 29 August 2019), 2011a. a
EOS MLS Science Team: MLS/Aura Level 2 Temperature V003, Goddard Earth Sciences Data and Information Services Center (GES DISC), Greenbelt, MD, USA, available at: https://disc.gsfc.nasa.gov/datacollection/ML2T_003.html (last access: 29 August 2019), 2011b. a
Funke, B., López-Puertas, M., Holt, L., Randall, C. E., Stiller, G. P., and von Clarmann, T.: Hemispheric distributions and interannual variability of NOy produced by energetic particle precipitation in 2002–2012, J. Geophys. Res.-Atmos., 119, 13565–13582, https://doi.org/10.1002/2014JD022423, 2014a. a, b, c, d, e, f, g, h, i, j, k
Funke, B., López-Puertas, M., Stiller, G. P., and von Clarmann, T.: Mesospheric and stratospheric NOy produced by energetic particle precipitation during 2002–2012, J. Geophys. Res.-Atmos., 119, 4429–4446, https://doi.org/10.1002/2013JD021404, 2014b. a, b, c, d, e
Funke, B., López-Puertas, M., Stiller, G. P., Versick, S., and von Clarmann, T.: A semi-empirical model for mesospheric and stratospheric NOy produced by energetic particle precipitation, Atmos. Chem. Phys., 16, 8667–8693, https://doi.org/10.5194/acp-16-8667-2016, 2016. a
Garcia, R. R. and Solomon, S.: A possible relationship between interannual variability in Antarctic ozone and the quasi-biennial oscillation, Geophys. Res. Lett., 14, 848–851, https://doi.org/10.1029/GL014i008p00848, 1987. a
Hurwitz, M. M., Newman, P. A., Li, F., Oman, L. D., Morgenstern, O., Braesicke, P., and Pyle, J. A.: Assessment of the breakup of the Antarctic polar vortex in two new chemistry-climate models, J. Geophys. Res.-Atmos., 115, D07105, https://doi.org/10.1029/2009JD012788, 2010. a
Jackman, C. H., DeLand, M. T., Labow, G. J., Fleming, E. L., Weisenstein, D. K., Ko, M. K. W., Sinnhuber, M., and Russell, J. M.: Neutral atmospheric influences of the solar proton events in October–November 2003, J. Geophys. Res.-Space, 110, A09S27, https://doi.org/10.1029/2004JA010888, 2005. a
Jackman, C. H., Marsh, D. R., Vitt, F. M., Garcia, R. R., Fleming, E. L., Labow, G. J., Randall, C. E., López-Puertas, M., Funke, B., von Clarmann, T., and Stiller, G. P.: Short- and medium-term atmospheric constituent effects of very large solar proton events, Atmos. Chem. Phys., 8, 765–785, https://doi.org/10.5194/acp-8-765-2008, 2008. a
Krotkov, N. A., Lamsal, L. N., Celarier, E. A., Swartz, W. H., Marchenko, S. V., Bucsela, E. J., Chan, K. L., Wenig, M., and Zara, M.: The version 3 OMI NO2 standard product, Atmos. Meas. Tech., 10, 3133–3149, https://doi.org/10.5194/amt-10-3133-2017, 2017. a
Krotkov, N. A., Lamsal, L. N., Marchenko, S. V., Celarier, E. A., Bucsela, E. J., Swartz, W. H., Joiner, J., and the OMI core team: OMI/Aura NO2 Total and Tropospheric Column Daily L2 Global Gridded 0.25 degree × 0.25 degree V3, NASA Goddard Space Flight Center, Goddard Earth Sciences Data and Information Services Center (GES DISC), https://doi.org/10.5067/Aura/OMI/DATA2018, 2019. a, b
Kuttippurath, J. and Nair, P.: The signs of Antarctic ozone hole recovery, Sci. Rep.-UK, 7, 585, https://doi.org/10.1038/s41598-017-00722-7, 2017. a
Lait, L. R., Schoeberl, M. R., and Newman, P. A.: Quasi-biennial modulation of the Antarctic ozone depletion, J. Geophys. Res.-Atmos., 94, 11559–11571, https://doi.org/10.1029/JD094iD09p11559, 1989. a
Livesey, N. J., Read, W. G., Wagner, P. A., Froidevaux, L., Lambert, A., Manney, G. L., Millán Valle, L. F., Hugh C. Pumphrey, H. C., Santee, M. L., Schwartz, M. J., Wang, S., Fuller, R. A., Jarnot, R. F., Knosp, B. W., Martinez, E., and Lay, R. R.: Earth Observing System (EOS) Aura Microwave Limb Sounder (MLS) version 4.2x level 2 data quality and description document, available at: https://mls.jpl.nasa.gov/data/v4-2_data_quality_document.pdf (last access: 30 August 2019), 2017. a
Manney, G., Santee, M., Froidevaux, L., Livesey, N., and Read, W.: MLS/Aura Level 2 Nitric Acid (HNO3) Mixing Ratio V004, Goddard Earth Sciences Data and Information center, https://doi.org/10.5067/Aura/MLS/DATA2012, 2015. a
Matthes, K., Funke, B., Andersson, M. E., Barnard, L., Beer, J., Charbonneau, P., Clilverd, M. A., Dudok de Wit, T., Haberreiter, M., Hendry, A., Jackman, C. H., Kretzschmar, M., Kruschke, T., Kunze, M., Langematz, U., Marsh, D. R., Maycock, A. C., Misios, S., Rodger, C. J., Scaife, A. A., Seppälä, A., Shangguan, M., Sinnhuber, M., Tourpali, K., Usoskin, I., van de Kamp, M., Verronen, P. T., and Versick, S.: Solar forcing for CMIP6 (v3.2), Geosci. Model Dev., 10, 2247–2302, https://doi.org/10.5194/gmd-10-2247-2017, 2017. a, b
Naujokat, B.: An Update of the Observed Quasi-Biennial Oscillation of the Stratospheric Winds over the Tropics, J. Atmos. Sci., 43, 1873–1877, https://doi.org/10.1175/1520-0469(1986)043<1873:AUOTOQ>2.0.CO;2, 1986. a
Randall, C. E., Rusch, D. W., Bevilacqua, R. M., Hoppel, K. W., and Lumpe, J. D.: Polar Ozone and Aerosol Measurement (POAM) II stratospheric NO2, 1993–1996, J. Geophys. Res.-Atmos., 103, 28361–28371, https://doi.org/10.1029/98JD02092, 1998. a, b
Randall, C. E., Harvey, V. L., Manney, G. L., Orsolini, Y., Codrescu, M., Sioris, C., Brohede, S., Haley, C. S., Gordley, L. L., Zawodny, J. M., and Russell, J. M.: Stratospheric effects of energetic particle precipitation in 2003–2004, Geophys. Res. Lett., 32, L05802, https://doi.org/10.1029/2004GL022003, 2005. a
Randall, C. E., Harvey, V. L., Singleton, C. S., Bailey, S. M., Bernath, P. F., Codrescu, M., Nakajima, H., and Russell, J. M.: Energetic particle precipitation effects on the Southern Hemisphere stratosphere in 1992–2005, J. Geophys. Res., 112, D08308, https://doi.org/10.1029/2006JD007696, 2007. a, b, c, d, e
Santee, M. L., Read, W. G., Waters, J. W., Froidevaux, L., Manney, G. L., Flower, D. A., Jarnot, R. F., Harwood, R. S., and Peckham, G. E.: Interhemispheric Differences in Polar Stratospheric HNO3, H2O, ClO, and O3, Science, 267, 849–852, https://doi.org/10.1126/science.267.5199.849, 1995. a
Santee, M. L., Lambert, A., Read, W. G., Livesey, N. J., Cofield, R. E., Cuddy, D. T., Daffer, W. H., Drouin, B. J., Froidevaux, L., Fuller, R. A., Jarnot, R. F., Knosp, B. W., Manney, G. L., Perun, V. S., Snyder, W. V., Stek, P. C., Thurstans, R. P., Wagner, P. A., Waters, J. W., Muscari, G., de Zafra, R. L., Dibb, J. E., Fahey, D. W., Popp, P. J., Marcy, T. P., Jucks, K. W., Toon, G. C., Stachnik, R. A., Bernath, P. F., Boone, C. D., Walker, K. A., Urban, J., and Murtagh, D.: Validation of the Aura Microwave Limb Sounder HNO3 measurements, J. Geophys. Res.-Atmos., 112, D24S40, https://doi.org/10.1029/2007JD008721, 2007. a
Schoeberl, M. R., Douglass, A. R., and Joiner, J.: Introduction to special section on Aura Validation, J. Geophys. Res.-Atmos., 113, D15S01, https://doi.org/10.1029/2007JD009602, 2008. a
Schwartz, M., L. N. and Read, W.: MLS/Aura Level 2 Temperature V004, Goddard Earth Sciences Data and Information center, https://doi.org/10.5067/Aura/MLS/DATA2021, 2015. a
Schwartz, M. J., Lambert, A., Manney, G. L., Read, W. G., Livesey, N. J., Froidevaux, L., Ao, C. O., Bernath, P. F., Boone, C. D., Cofield, R. E., Daffer, W. H., Drouin, B. J., Fetzer, E. J., Fuller, R. A., Jarnot, R. F., Jiang, J. H., Jiang, Y. B., Knosp, B. W., Krüger, K., Li, J.-L. F., Mlynczak, M. G., Pawson, S., Russell III, J. M., Santee, M. L., Snyder, W. V., Stek, P. C., Thurstans, R. P., Tompkins, A. M., Wagner, P. A., Walker, K. A., Waters, J. W., and Wu, D. L.: Validation of the Aura Microwave Limb Sounder temperature and geopotential height measurements, J. Geophys. Res.-Atmos., 113, D15S11, https://doi.org/10.1029/2007JD008783, 2008. a
Seppälä, A., Verronen, P. T., Clilverd, M. A., Randall, C. E., Tamminen, J., Sofieva, V., Backman, L., and Kyölä, E.: Arctic and Antarctic polar winter NOx and energetic particle precipitation in 2002–2006, Geophys. Res. Lett., 34, L12810, https://doi.org/10.1029/2007GL029733, 2007a. a, b, c
Seppälä, A., Clilverd, M. A., and Rodger, C. J.: NOx enhancements in the middle atmosphere during 2003–2004 polar winter: Relative significance of solar proton events and the aurora as a source, J. Geophys. Res., 112, D23303, https://doi.org/10.1029/2006JD008326, 2007b. a
Seppälä, A., Matthes, K., Randall, C. E., and Mironova, I. A.: What is the solar influence on climate? Overview of activities during CAWSES-II, Prog. Earth Planet. Sci., 1, 24, https://doi.org/10.1186/s40645-014-0024-3, 2014. a
Siskind, D. E., Nedoluha, G. E., Randall, C. E., Fromm, M., and Russell III, J. M.: An assessment of Southern Hemisphere stratospheric NOx enhancements due to transport from the upper atmosphere, Geophys. Res. Lett., 27, 329–332, https://doi.org/10.1029/1999GL010940, 2000. a, b, c
Solomon, S., Ivy, D. J., Kinnison, D. E., Mills, M. J., Neely, R. R., and Schmidt, A.: Emergence of healing in the Antarctic ozone layer, Science, 353, 269–274, https://doi.org/10.1126/science.aae0061, 2016. a
Stone, K. A., Solomon, S., and Kinnison, D. E.: On the Identification of Ozone Recovery, Geophys. Res. Lett., 45, 5158–5165, https://doi.org/10.1029/2018GL077955, 2018. a
Strahan, S. E., Oman, L. D., Douglass, A. R., and Coy, L.: Modulation of Antarctic vortex composition by the quasi-biennial oscillation, Geophys. Res. Lett., 42, 4216–4223, https://doi.org/10.1002/2015GL063759, 2015. a, b, c, d, e, f, g
Turunen, E., Verronen, P. T., Seppälä, A., Rodger, C. J., Clilverd, M. A., Tamminen, J., Enell, C. F., and Ulich, T.: Impact of different energies of precipitating particles on NOx generation in the middle and upper atmosphere during geomagnetic storms, J. Atmos. Sol.-Terr. Phy., 71, 1176–1189, https://doi.org/10.1016/j.jastp.2008.07.005, 2009. a
Wilks, D. S.: Statistical methods in the atmospheric sciences, vol. 100, Elsevier, Oxford, UK, 2011. a
World Data Center for Geomagnetism: Kyoto, Operated by Kyoto University, available at: http://wdc.kugi.kyoto-u.ac.jp/kp, last access: 22 January 2019. a