the Creative Commons Attribution 4.0 License.
the Creative Commons Attribution 4.0 License.
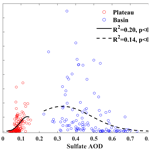
Distinct aerosol effects on cloud-to-ground lightning in the plateau and basin regions of Sichuan, Southwest China
Pengguo Zhao
Hui Xiao
Fang Wu
Youtong Zheng
Maureen C. Cribb
Xiaoai Jin
Yunjun Zhou
The joint effects of aerosol, thermodynamic, and cloud-related factors on cloud-to-ground lightning in Sichuan were investigated by a comprehensive analysis of ground-based measurements made from 2005 to 2017 in combination with reanalysis data. Data include aerosol optical depth, cloud-to-ground (CG) lightning density, convective available potential energy (CAPE), mid-level relative humidity, lower- to mid-tropospheric vertical wind shear, cloud-base height, total column liquid water (TCLW), and total column ice water (TCIW). Results show that CG lightning density and aerosols are positively correlated in the plateau region and negatively correlated in the basin region. Sulfate aerosols are found to be more strongly associated with lightning than total aerosols, so this study focuses on the role of sulfate aerosols in lightning activity. In the plateau region, the lower aerosol concentration stimulates lightning activity through microphysical effects. Increasing the aerosol loading decreases the cloud droplet size, reducing the cloud droplet collision–coalescence efficiency and inhibiting the warm-rain process. More small cloud droplets are transported above the freezing level to participate in the freezing process, forming more ice particles and releasing more latent heat during the freezing process. Thus, an increase in the aerosol loading increases CAPE, TCLW, and TCIW, stimulating CG lightning in the plateau region. In the basin region, by contrast, the higher concentration of aerosols inhibits lightning activity through the radiative effect. An increase in the aerosol loading reduces the amount of solar radiation reaching the ground, thereby lowering the CAPE. The intensity of convection decreases, resulting in less supercooled water being transported to the freezing level and fewer ice particles forming, thereby increasing the total liquid water content. Thus, an increase in the aerosol loading suppresses the intensity of convective activity and CG lightning in the basin region.
- Article
(8210 KB) - Full-text XML
-
Supplement
(614 KB) - BibTeX
- EndNote
Aerosol–cloud–precipitation interactions are complicated and are mainly reflected in the influence of aerosols on cloud microphysical and radiation processes, i.e., aerosol–cloud interactions (ACI) and aerosol–radiation interactions (ARI) (Rosenfeld et al., 2008; Huang et al., 2009; Koren et al., 2014; Li et al., 2011, 2017, 2019; Oreopoulos et al., 2020). The aerosol microphysical effect refers to the role of aerosols as cloud condensation nuclei (CCN) and ice nuclei (IN), influencing the microphysical processes of liquid- and ice-phase clouds. The aerosol radiation effect refers to the absorption and scattering of solar radiation by aerosols, changing the radiation balance between the atmosphere and the surface. The microphysical and radiative effects of aerosols combined with dynamic processes influence weather and climate processes through their links with meteorological conditions.
Lightning activity is mainly affected by atmospheric thermodynamic conditions and is an important indicator of the development of convective systems. The collision and separation of large and small ice particles mainly cause electrification. Supercooled water, ice particles, and strong updrafts are the components needed for the occurrence and development of lightning (MacGorman et al., 2001; Mansell et al., 2005; Williams, 2005; Price, 2013; Q. Wang et al., 2018; Qie and Zhang, 2019).
The differences in thermal conditions and aerosol loading between land and ocean areas lead to a higher lightning frequency over land than over oceans (Williams and Stanfill, 2002; Williams et al., 2004). Lightning activity over cities with higher aerosol concentrations is more intense than that over clean suburbs (Westcott, 1995; Pinto et al., 2004; Kar et al., 2009; Kar and Liou, 2014; Proestakis et al., 2016; Yair, 2018; Tinmaker et al., 2019). An increase in the aerosol concentration leads to the formation of more small cloud droplets, which have difficulty forming raindrops due to their low collision–coalescence efficiency, thereby inhibiting the warm-rain process. These small cloud droplets are transported above the freezing level, increasing the supercooled water content in a thunderstorm and significantly enhancing the ice-phase process. The freezing process releases more latent heat to stimulate convection, allowing more ice particles to participate in the electrification process of collision and separation, thereby enhancing lightning activity (Khain et al., 2008; Mansell and Ziegler, 2013; P. Zhao et al., 2015; Shi et al., 2015). A similar enhancement in lightning activity due to aerosols was also found in oceanic regions, where aerosols and their precursors discharged by ships significantly enhanced lightning activity over shipping lanes (Thornton et al., 2017). The influence of aerosols on thunderstorms is not linear. When the aerosol optical depth (AOD) is less than 0.3, aerosols can stimulate lightning activity. However, the intensity of the lightning activity will be inhibited if the concentration of aerosols increases above this level (Altaratz et al., 2010; Stallins et al., 2013; X. Li et al., 2018; Q. Wang et al., 2018).
The effect of aerosols on convective clouds and lightning activity is not only controlled by environmental factors but also by aerosol type. Absorbing aerosols in the boundary layer warm the atmosphere and cool the surface, which leads to an increase in the atmospheric convective inhibition energy and a rise in the convection condensation level (CCL); meanwhile, the absorbing aerosols also lead to an increase in the convective available potential energy above the CCL. Once the lifting condition overcomes the convective inhibition energy, strong convective activity will be triggered (Wang et al., 2013). Hygroscopic aerosols can stimulate the development of thunderstorms through microphysical effects under the appropriate environmental conditions (Wang et al., 2018). In central China, aerosol absorption of solar radiation has increased the stability of the lower atmosphere, reducing thunderstorm activity by 50 % from 1961 to 2000 (Yang et al., 2013). In Nanjing in eastern China, aerosols have reduced the amount of solar radiation reaching the surface and the convective available potential energy (CAPE), inhibiting the intensity of lightning activity (Tan et al., 2016). In the Sichuan Basin, with its complex topography, the influence of absorbing aerosols on strong convection is more complicated. During the day, aerosols absorb solar radiation and increase the stability of the lower atmosphere, accumulating a large amount of water vapor and energy in the basin. Under the influence of the uplift of the mountain terrain at night, convection is excited, and stronger convective precipitation is formed in the mountainous area (Fan et al., 2015). In southeast China where the hygroscopicity of aerosols dominates, an increase in aerosols in the plain areas significantly stimulates lightning activity (Yuan et al., 2011; Wang et al., 2011), whereas the influence of aerosols on thunderstorms in mountainous areas with slightly higher altitudes is not prominent (Yang and Li, 2014). Aerosol radiative and microphysical effects have different impacts on thunderstorms at different stages of their development. In the Pearl River Delta region, the daytime radiative effect delays lightning activity, whereas the aerosol microphysical effect at night further stimulates lightning activity (Guo et al., 2016; Lee et al., 2016).
Sichuan Province is located in Southwest China, with the Tibetan Plateau and the Hengduan Mountains to the west, the Qinba Mountains to the north, and the Yunnan–Guizhou Plateau to the south. The western part of Sichuan Province is dominated by plateau and mountainous terrain, with an average elevation of about 2000 to 4000 m, whereas the eastern part is dominated by a basin and hilly terrain, with an average elevation of 300 to 700 m. The thermal and moisture conditions in the basin facilitate lightning activity (Xia et al., 2015; Yang et al., 2015). The Sichuan Basin is an area with a high aerosol loading and with complex terrain not conducive to pollutant dispersion (Zhang et al., 2012; Sun et al., 2016; Wei et al., 2019a, b; Ning et al., 2018a; 2019).
There are significant geographical and environmental differences between the western Sichuan Plateau and the eastern Sichuan Basin. The thermal conditions of the western Sichuan Plateau are obviously weaker than those of the Sichuan Basin (Qie et al., 2003), and the aerosol concentration in the plateau is also significantly lower than that in the basin (Ning et al., 2018a). Previous studies (Yuan et al., 2011; Wang et al., 2011; Yang and Li, 2014; Fan et al., 2015; Yang et al., 2016) have suggested that aerosol effects on lightning activity differ significantly due to differences in topography and aerosol. The purpose of this study is to investigate any similarities and differences in the effects of aerosols on lightning activity in the context of different topography and aerosol concentrations between the western Sichuan Plateau and Sichuan Basin.
In this study, we investigate the joint effects of aerosol, thermodynamic, and cloud-related conditions on cloud-to-ground (CG) lightning activity under such special topographic conditions. This paper is organized as follows: Sect. 2 describes the data and methodology used in the study, Sect. 3 presents and discusses the results, and Sect. 4 summarizes the study.
2.1 CG lightning
Hourly CG lightning flash data from 2005 to 2017 were obtained from the Sichuan Meteorological Bureau. CG lightning flashes are observed by the Sichuan Lightning Detection Network (SCLDN), which belongs to the Lightning Detection Network of the China Meteorological Administration (CMA), and consists of 25 detection sensors (Fig. 1). The average detection accuracy of the sensor is ∼300 m, the average detection radius is 300 km, and the detection efficiency is 80 %–90 % (Yang et al., 2015). The SCLDN is based on the ground-based Advanced Time of Arrival and Direction system, which uses improved accuracy from the combined technology method (Cummins et al., 1998; CMA, 2009).
Positive CG lightning flashes with peak currents less than 15 kA are removed to avoid the contamination of cloud-to-cloud lightning (Cummins and Murphy, 2009). A flash is identified if the location of the first stroke is within 10 km and the time interval between two contiguous strokes is less than 0.5 s. If the polarity of the stroke is different, it is a different flash (Cummins et al., 1998). To match the thermodynamic and cloud-related parameters, the CG lightning data used in this study were calculated at a 0.25∘ horizontal resolution. Many previous studies (e.g., Orville et al., 2011; Ramos et al., 2011; Yang et al., 2015) have also discussed the basic characteristics of lightning at a similar resolution.
2.2 AOD
The Modern-Era Retrospective analysis for Research and Applications, version 2 (MERRA-2), data set provided AODs from 2005 to 2017. The quality-controlled MERRA-2 AOD product (at 550 nm) provides the optical thicknesses of different types of aerosols, including total aerosol, sulfate, black carbon, organic carbon, and dust, with a spatial resolution of (Randles et al., 2017; Buchard et al., 2017). To match CG lightning data, we interpolated AOD data onto the same 0.25∘ spatial resolution grid. The horizontal distribution and vertical structure of MERRA-2 aerosol optical properties are in good agreement with satellite and aircraft observations (Buchard et al., 2017). Sun et al. (2019a, b) employed MODerate resolution Imaging Spectroradiometer (MODIS) and Aerosol Robotic Network (AERONET) AOD products to evaluate the MERRA-2 AOD over China. They reported that the MERRA-2 and MODIS AODs agreed well and that the seasonal correlation coefficients between the MERRA-2 and AERONET AODs ranged from 0.87 to 0.92. Figure S1 in the Supplement shows the spatial distribution of AOD based on the monthly data of MERRA-2 and Multi-angle Imaging SpectroRadiometer (MISR) data sets from 2005 to 2017. It can be seen from Fig. S1 that the AOD spatial distribution of MISR is very close to that of MERRA-2, but the AOD value of MISR is smaller than that of MERRA-2. Wei et al. (2019c) suggested that there is a good consistency between MISR and MODIS AOD products in Southwest China using multi-satellite data comparison.
2.3 Thermodynamic and cloud-related parameters
Thermodynamic and cloud-related factors include CAPE, mid-level relative humidity (RH), lower- to mid-tropospheric vertical wind shear (SHEAR), cloud-base height (CBH), total column liquid water (TCLW), and total column ice water (TCIW), collected from ERA5 reanalysis data with a spatial resolution of (Dee et al., 2011).
Hoffmann et al. (2019) indicated that the ERA5 reanalysis is more representative of atmospheric convection, mesoscale cyclones, and mesoscale to synoptic-scale atmospheric characteristics than the earlier ERA-Interim reanalysis. Freychet et al. (2020) found that the dry-bulb temperature, wet-bulb temperature, and RH of the ERA5 reanalysis were representative through comparisons with ground-based observations made in China. Lee et al. (2018) compared the water vapor and liquid water distributions observed by a microwave radiometer in Seoul, South Korea, with that of the ERA5 reanalysis and found that they agreed well. Shou et al. (2019) confirmed that ERA5 data captured the cloud-top features based on multi-satellite observations made over the Tibetan Plateau. Zhang et al. (2019) pointed out that the ERA5 precipitable water vapor field agreed well with radiosonde and Global Navigation Satellite System observations. Lei et al. (2020) examined the representation of ERA5 cloud-cover characteristics over China through comparisons with satellite observations, reporting that (1) ERA5 overestimated the cloud cover by ∼10 %, and (2) the long-term trend in ERA5 cloud cover was consistent with satellite observations. These studies suggest that ERA5 cloud-related data from China have sound quality. We compared the liquid water paths (LWPs) and ice water paths (IWPs) of CLARA-A2 and ERA5 data sets. The monthly CLARA-A2 record, with a spatial resolution of , provides cloud properties, surface albedo, and surface radiation parameters derived from the Advanced Very High-Resolution Radiometer (AVHRR) sensor (Karlsson et al., 2017a; Karlsson and Håkansson, 2018). Overall, the cloud products of the two data sets were similar (Fig. S2 in the Supplement). For the continuity of data, LWP and IWP in ERA5 were selected in this study.
CAPE is the most important factor controlling lightning, and climate projections suggest that an increase in the CAPE caused by global warming could increase global lightning by 50 % in the 21st century (Romps et al., 2014). The proxy composed of precipitation rate and CAPE has a good correlation with observed lightning density over the United States (Romps et al., 2018; Tippett and Koshak, 2018; Tippett et al., 2019). CAPE is the factor with the highest relative contribution in various lightning parameterization schemes (Bang and Zipser, 2016; Stolz et al., 2015, 2017).
Due to the large elevation fluctuation in Sichuan, pressure-level data are not applicable for the analysis of the atmospheric vertical structure. Thus, pressure levels were changed to geometric altitudes above ground level, using the following barometric formula (Minzner, 1977):
where Z2 and Z1 are the elevations of the two isobaric levels (in m), P2 and P1 are the pressures of the two isobaric levels (in hPa), P1 is 1000 hPa, Z1 is 0 m, and ta is the average temperature of the two isobaric levels (in ∘). The elevation minus topographic height is the altitude above ground level:
where H is the geometric altitude above ground level, and Ht is the topographic height.
The mid-level RH and the lower- to mid-tropospheric SHEAR are important humidity and dynamic parameters, directly affecting the formation, development, propagation, and intensity of thunderstorms (Davies-Jones, 2002; Thompson et al., 2007; Wall et al., 2014; Bang and Zipser, 2016). In this study, RH is the average RH in the 3–5 km layer, and SHEAR is the vertical wind shear in the 0–5 km layer:
where u2, u1, v2, and v1 are zonal and meridional wind speeds at 5 and 3 km, respectively.
CBH, TCLW, and TCIW were selected to represent cloud-related parameters affecting the development of lightning activity. CBH, which is negatively correlated with the warm-cloud thickness, controls the convective structure and the polarity and intensity of CG lightning by affecting the liquid water and ice water contents (Williams et al., 2005; Carey and Buffalo, 2007; Stolz et al., 2017). Liquid water and ice water, especially in the noninductive electrification zone, directly control the processes of charge generation and separation that determine the intensity of lightning of a thunderstorm (Yair et al., 2010; Wong et al., 2013; Dafis et al., 2018).
In this study, we use the Pearson correlation and partial correlation to discuss the relationship between two elements at each grid point. Data from 156 months during the 2005–2017 period were used, and monthly averages were calculated. Data at each grid point were processed using a three-point moving average. Table 1 shows the acronyms of variables used in this study.
3.1 Distributions of CG lightning and AOD
Due to the complex terrain in Sichuan, the CG lightning density and AOD differ greatly across the province. The CG lightning density is highest over the basin region in eastern Sichuan, with an annual average density of 1–3 (Fig. 2a). The lightning density in western Sichuan is much lower than that in the basin region. Yang et al. (2015) showed that the Sichuan Basin is one of the most CG-lightning-active regions in China, besides the Yangtze River Delta and the Pearl River Delta. The dramatic difference in lightning density between the basin and the plateau stems primarily from differences in humidity and thermal conditions. Another factor is the generation of strong convective systems caused by the eastward migration of the southwest vortex formed over the Tibetan Plateau to the basin area (Yu et al., 2007; Zhang et al., 2014). The total AOD over the basin region is significantly higher than that over the plateau region. The mean AOD over the basin is about 0.6–0.9, whereas that over the plateau is about 0.15 (Fig. 2b). The aerosols in Sichuan are mainly composed of sulfate aerosols, accounting for about 60 %–80 % of the total AOD over the basin and 40 %–55 % of the total AOD over the plateau (Fig. 2d). Aerosol concentrations over the basin are higher than those over the plateau area, mainly because of the greater amount of anthropogenic air pollutants emitted in the basin (Zhang et al., 2012). Also playing important roles are the mountains around the basin and the low-pressure system at 700 hPa over the basin, resulting in a strong inversion above the planetary boundary layer (Ning et al., 2018b).
3.2 Correlation between AOD and CG lightning
While the spatial patterns of lightning intensity (Fig. 2a) and AOD (Fig. 2b) bear some resemblance, one cannot draw a straight conclusion that the latter is the cause of the former because they are both affected by the topography. However, the influences of aerosols on lightning have been well established in previous studies by affecting the local meteorological environment through aerosol radiative and microphysical effects (Yang et al., 2013; Q. Wang et al., 2018; Z. Li et al., 2019). To circumvent the topographic influence, Fig. 3 shows the Pearson correlation coefficients of total AOD and sulfate AOD as well as CG lightning density in individual grid boxes in Sichuan. It is interesting to note that the correlation between aerosol loading and lightning is the inverse in the plateau region compared with the basin region, i.e., a positive correlation in the plateau region and a negative correlation in the basin region. This suggests that aerosols stimulate lightning in the plateau region but suppress lightning in the basin region. Such a distinct difference may be related to differences in aerosol loading and local environmental factors (Rosenfeld et al., 2007; Fan et al., 2009; Carrió and Cotton, 2014). The maximum value of the positive correlation coefficient was about 0.5, occurring in the plateau region of central Sichuan. The maximum values of the negative correlation coefficients occurred in the basin region of eastern Sichuan. The absolute values of the negative correlation coefficients are larger than those of the positive correlation coefficients. The distribution of the correlation coefficients between lightning and sulfate AOD is similar to that of total AOD, but there are more and larger positive correlation coefficients than negative ones. As sulfate AOD accounts for about 60 %–80 % of the total AOD over the basin region and 40 %–55 % of the total AOD over the plateau region, this study mainly discusses the relationship between sulfate AOD and lightning activity. The plateau and basin regions in this study are outlined in Fig. 3b to discuss the effects of sulfate aerosols on lightning activity in the two regions separately.
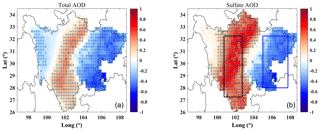
Figure 3Pearson correlation coefficients between total AOD and CG lightning (a) and sulfate AOD and CG lightning (b) based on monthly data from 2005 to 2017. The correlation coefficient of each grid box is calculated from 156 monthly average data sets, and monthly average data are processed using a three-point moving average. Crosses in the figure indicate grid boxes that have passed the 95 % significance test. The plateau region and the basin region are outlined by black and blue rectangles, respectively, in panel (b).
To further verify the stimulation and inhibition of aerosols on lightning activity and eliminate the interference of seasonality on the effects of aerosols on lightning, Pearson correlation coefficients between anomalies of total AOD and CG lightning and anomalies of sulfate AOD and CG lightning were implemented. As can be seen from the comparison between Figs. 3 and 4, the correlation coefficients between the anomalies of AOD and lightning are significantly lower than those between AOD and lightning. However, in an overall view, there is still a positive correlation between aerosols and lightning in the plateau region and a negative correlation between aerosols and lightning in the basin region, especially for sulfate aerosols. This further verifies that aerosols have the potential to stimulate lightning activity in the plateau region and inhibit lightning activity in the basin region. The specific physical relationship will be further discussed below.
Note that a statistical relationship between two variables does not necessarily imply a true causality between the two; to demonstrate causality, further insights would be needed. The spatial contrast exhibited in the correlation maps, however, conveys valuable information about the causality, because the influences of large-scale meteorology may have little to do with the spatial pattern.
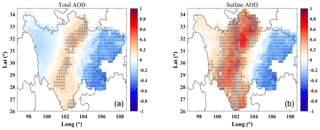
Figure 4Pearson correlation coefficients between anomalies of total AOD and CG lightning (a) and anomalies of sulfate AOD and CG lightning (b) based on monthly data from 2005 to 2017. Crosses in the figure indicate grid boxes that have passed the 90 % significance test.
To further analyze the relationship between aerosols and lightning over Sichuan, Fig. 5 shows the CG lightning density as a function of sulfate AOD over the plateau and basin regions. Due to differences in emissions, the aerosol loading over the plateau region is much lighter than that over the basin region. The regional average sulfate AOD over the plateau region ranges from 0.03 to 0.15, and that over the basin region ranges from 0.22 to 0.87. The difference in CG lightning density is mainly related to the different meteorological conditions of the plateau and the basin. The monthly regional average CG lightning density over the plateau is to 0.35 flashes km−2, whereas that over the basin is to 0.85 flashes km−2. In the plateau region, the lightning density increases exponentially with increasing AOD, whereas in the basin region, the lightning density decreases exponentially with increasing AOD. This difference may be due to the different microphysical and radiative effects of different aerosol loadings. Previous studies (Koren et al., 2008, 2012; Altaratz et al., 2010, 2017; Liu et al., 2019) have noted a turning point of AOD=0.3 with regard to the influence of AOD on clouds. For lower AOD, aerosols can stimulate lightning activity through microphysical effects. For higher AOD, aerosols reduce the solar radiation reaching the surface through the radiative effect, thereby inhibiting lightning activity.
3.3 Correlation between thermodynamic and cloud-related factors and CG lightning
Thermodynamic and cloud-related parameters are the decisive factors determining the occurrence and development of lightning activity (Williams, 2005; Williams et al., 2005; Saunders, 2008; Stolz et al., 2017). Figure 6 shows the respective correlation coefficients of CAPE, RH, SHEAR, CBH, TCLW, and TCIW with CG lightning density over Sichuan. The thermodynamic parameters CAPE and RH, especially CAPE, have significant excitation effects on lightning activity, whereas SHEAR shows a significant negative correlation with lightning. There is a positive correlation between TCIW and lightning density over Sichuan because the development of lightning mainly depends on the noninductive electrification of the collision and separation of large and small ice particles. The more ice particles, the stronger the lightning activity will be. The correlation between CBH and lightning is the inverse of that between TCLW and lightning in the plateau and basin regions. Over the plateau area, low cloud bases and high liquid water contents are favorable for lightning activity, whereas the opposite is seen over the basin. A higher CBH means that the warm-cloud depth is thinner, so the liquid water content will be less. In the plateau region, because of the compression effect of the plateau topography on clouds, the warm-cloud depth is much thinner than that in the basin region. Increasing a fixed amount of liquid water is conducive to transporting supercooled water to the upper layer and promoting the development of the ice-phase process. The more vigorous the ice-phase process is, the more intense the lightning activity will be. Over the basin, where warm clouds are thicker, an increase in liquid water will more likely promote the development of the warm-rain process rather than the ice-phase process. Figure S3 in the Supplement shows the correlation coefficients between the anomalies of CAPE, RH, SHEAR, CBH, TCLW, and TCIW with CG lightning. Compared with Fig. 6, the correlation coefficients are obviously smaller, especially in the basin region. The significance of the correlation between CG lightning and environmental factors are weakened, especially for SHEAR, CBH, and TCLW in the basin region.
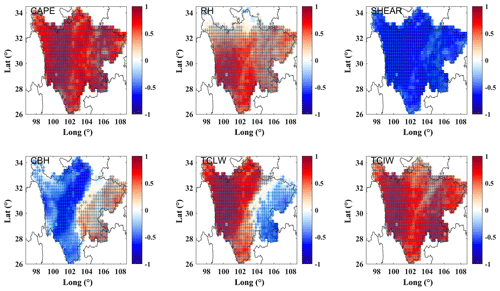
Figure 6Pearson correlation coefficients of CAPE, RH, SHEAR, CBH, TCLW, and TCIW with CG lightning. Crosses in the figure indicate grid boxes that have passed the 95 % significance test.
Partial correlation analysis has been used in many previous studies (Wang et al., 2018; Zhao et al., 2019) to discuss the dependence between two variables when influence from other variables is possible. To avoid interactions between the factors involved and to discuss the relationships between different factors and lightning activity more independently, Fig. 7 shows the partial correlation coefficients of thermodynamic and cloud-related parameters with CG lightning density. In terms of the thermodynamic parameters, the partial correlation coefficients show that the dependence of lightning on RH and SHEAR is not significant. The partial correlation coefficient of some regions in Sichuan is zero. Compared with RH, the absolute value of the negative partial correlation coefficient of SHEAR is larger and more widely distributed, indicating that SHEAR has a more significant impact (inhibition) on lightning activity than RH does. CAPE is positively correlated with lightning in Sichuan, and the partial correlation coefficient of many grid points is greater than 0.2, indicating that CAPE is a crucial factor controlling lightning, as reported by others (Carey and Buffalo, 2007; Fuchs et al., 2015; Bang and Zipser, 2016; Stolz et al., 2017). Among the cloud-related parameters, the partial correlation coefficients of CBH and TCLW with lightning are lower, indicating that CBH and TCLW have less significant influences on lightning density. The existence of supercooled water is one of the essential conditions for the electrification of thunderstorms. The supercooled liquid water content in different temperature ranges can affect the polarity of the charge carried by ice particles but cannot directly affect the intensity of the electrical activity of thunderstorms (Saunders et al., 1991; Saunders, 2008). The positive partial correlation coefficient between TCLW and lightning is relatively higher, especially in the basin area, indicating that ice particles, as the carrier of charge, can directly determine the occurrence and development process of lightning activity.
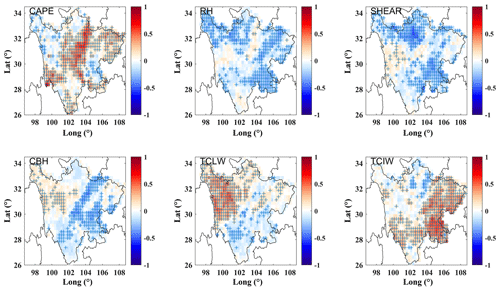
Figure 7Partial correlation coefficients of CG lightning with thermodynamic and cloud-related factors, i.e., CAPE, RH, SHEAR, CBH, TCLW, and TCIW. Crosses in the figure indicate grid boxes that have passed the 95 % significance test.
To demonstrate the differences in thermodynamic and cloud-related factors between the plateau and basin regions, Fig. 8 shows CG lightning density as a function of the thermodynamic and cloud-related parameters in the plateau and basin regions, based on monthly regionally averaged data. There is a significant positive correlation between CAPE and CG lightning density in both the plateau and basin regions, with a coefficient of determination (R2) of 0.53 and 0.51, respectively. CAPE over the plateau region is much smaller than that over the basin region. The maximum CAPE over the plateau area is , whereas the maximum CAPE over the basin area is greater 1000 J kg−1. This is the main reason why the CG lightning density over the basin region is larger than that over the plateau region. RH and CG lightning density were positively correlated in both plateau and basin regions, although not significantly in the basin region (R2=0.08). Due to the high altitude of the plateau and strong wind speeds there, SHEAR in the plateau region (maximum value of 40 m s−1) is significantly larger than that in the basin region (maximum value of 15 m s−1). The greater mid-level wind shear over the plateau region suppresses the intensity of lightning activity.
Due to the compression of clouds by the plateau topography, the mean CBH over the plateau region is relatively low, about 500–2000 m, whereas the mean CBH over the basin region is about 1000–3500 m. The correlation between CBH and lightning density is negative in the plateau. In the basin, however, there is barely any correlation (R2=0.02). The much lower temperature over the plateau directly results in a lower liquid water content there. The maximum value of TCLW is , whereas that in the basin region is . Correlations in the plateau region are more significant than in the basin region, with an R2 value of 0.26 and 0.19, respectively. The TCIW values over the plateau and basin areas are similar in magnitude. The positive correlation between TCIW and lightning density is also significant, with an R2 value of 0.34 and 0.42 in the basin and plateau regions, respectively. Except for the correlation between CBH and lightning in the basin region, the linear correlations between the other factors and lightning passed the 95 % significance test.
3.4 Joint effects of thermodynamic and cloud-related factors and aerosols on CG lightning
Based on the partial correlation and linear fitting analyses, CAPE, SHEAR, TCLW, and TCIW are the main thermodynamic and cloud-related factors controlling CG lightning over the Sichuan region. To analyze the joint effects of thermodynamic factors, Figs. 9 and 10 show scatterplots of sulfate AOD, CAPE, SHEAR, TCLW, and TCIW with CG lightning density in the plateau and basin regions. In the plateau region (Fig. 9), increases in CAPE, TCLW, and TCIW enhance lightning activity. As discussed before (Fig. 7), strong convective activity and more liquid water and ice water indicate that strong updrafts transport a greater amount of liquid-phase and ice-phase particles to the electrification area to participate in the electrification process, generating stronger lightning activity. Aerosol excitation of lightning may be achieved by increasing CAPE, TCLW, and TCIW. In the case of low aerosol loading, through ACI, an increase in aerosols will reduce the size of cloud droplets and increase the concentration of cloud droplets (Khain et al., 2008; Qian et al., 2009). Smaller cloud droplets reduce the collision–coalescence efficiency and inhibit the warm-rain process. Small cloud droplets that do not fall are transported above the freezing layer to participate in the freezing process and release more latent heat. This is consistent with previous studies (Mansell and Ziegler, 2013; P. Zhao et al., 2015; Altaratz et al., 2017; Fan et al., 2018; C. Zhao et al., 2018) and explains the potential cause of the increase in aerosols, leading to an increase in liquid water and ice water in thunderstorms, promoting convective activities. From the joint influence of CAPE, SHEAR, TCLW, and TCIW on lightning activity (Fig. 9d, e, f), an increase in CAPE inhibits the vertical wind shear in the lower to middle troposphere (Li et al., 2013; Sherburn et al., 2016), which is conducive to the development of lightning activity. Increasing CAPE also suggests that strong updrafts promote the development of convection, resulting in the formation of more liquid water and ice water in the cloud.
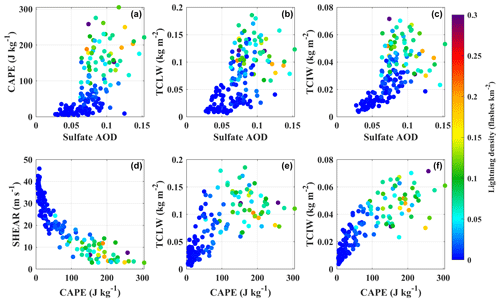
Figure 9Joint effects of sulfate AOD and CAPE (a), sulfate AOD and TCLW (b), sulfate AOD and TCIW (c), CAPE and SHEAR (d), CAPE and TCLW (e), and CAPE and TCIW (f) on CG lighting density over the plateau region. The color of the dots represents the CG lightning density.
The aerosol loading over the basin region is much higher than that over the plateau region, with sulfate AODs ranging from 0.2 to 0.9 (Fig. 10). Excessive aerosol loading inhibits convective development through ARI. Aerosols reduce the solar radiation reaching the surface through absorption and scattering, decreasing the convective energy of the surface and the lower atmosphere (Zhao et al., 2006; Jiang et al., 2018). Thus, weak updrafts cannot transport liquid water above the freezing level. This may be why the increase in aerosols leads to an increase in liquid water content and a decrease in ice water content. Aerosols reduce the intensity of lightning activity by inhibiting the development of convection and the formation of ice particles. This has also been observed in other regions (Yang et al., 2013; Tan et al., 2016). CAPE is higher over the basin region than over the plateau region (Fig. 10d, e, f). An increase in CAPE leads to a decrease in SHEAR and an increase in the ice water content, promoting the development of lightning, similar to the plateau region. Fan et al. (2009) found that under large vertical wind shear conditions, an increase in aerosols inhibits the development of convection. However, when CAPE exceeded 300 J kg−1, an increase in CAPE led to a decrease in the liquid water content. Convective clouds over the basin are thicker than those over the plateau, and the high CAPE makes convection develop more vigorously. In this way, liquid water is transported above the freezing level to participate in the ice-phase process, forming more ice particles.
According to the above, we hypothesize that the microphysical effect of aerosols is responsible for stimulating lightning activity over the plateau region and that the radiative effect of aerosols is responsible for suppressing lightning activity over the basin region. The radiative effect of aerosols impacts lightning by affecting CAPE, whereas the microphysical effect of aerosols impacts lightning by affecting the liquid water and ice water contents. To further verify the radiative effect of aerosols in the basin and the microphysical effect of aerosols in the plateau, two lightning sensitivity parameters are employed:
where RLr is a relative measure of lightning sensitivity to the effect of CAPE, associated with the aerosol radiative effect, and FC is the CG lightning flash count. Tinmaker et al. (2019) evaluated the impact of CAPE on lightning over land and oceanic regions using FC∕CAPE:
where RLm is a relative lightning parameter accounting for the effect of TCLW and TCIW on lightning, associated with the aerosol microphysical effect. As CAPE is an essential factor for generating lightning, it is also considered in this formulation.
Figure 11 shows the Pearson correlation coefficients between RLr, RLm, and sulfate AOD over Sichuan. Compared with the correlation between sulfate AOD and CG lightning (Fig. 3b), the negative correlation between sulfate AOD and RLr decreased significantly in the basin area, especially in the northern part of the basin, whereas the positive correlation between AOD and RLr did not change significantly in the plateau region. This suggests that the inhibitory effect of aerosols on lightning in the basin region is dependent on the effect on CAPE, but this is not the case in the plateau region, which also reflects the significant radiative effect of aerosols in the basin region. By comparing the correlation between sulfate AOD and RLm (Fig. 11b) and the correlation between sulfate AOD and CG lightning (Fig. 3b), the positive correlation coefficients between sulfate AOD and RLm in the plateau region decreased significantly, indicating that aerosols in the plateau region have a significant microphysical effect, stimulating the development of lightning activity by influencing liquid- and ice-phase particles in thunderstorms.
3.5 Multiple linear regression of CG lightning
Because the physical processes involved in the development of lightning are complex, many previous studies (e.g., Allen and Pickering, 2002; Tippett and Koshak, 2018) have parameterized lightning in weather and climate models using statistical regression methods instead of describing the specific physical processes of lightning in the model. Stolz et al. (2017) developed a global lightning parameterization scheme based on multiple linear regression, combining aerosol and thermodynamic parameters, which explained 69 %–81 % of the lightning activity in tropical and subtropical regions. The multiple linear regression equations are based on the least-squares method and monthly regionally averaged data. As there is little or no lightning activity in winter, January, February, and December are excluded. For the plateau region,
and for the basin region,
where Y is the CG lightning density, x1 is CAPE, x2 is RH, x3 is SHEAR, x4 is CBH, x5 is TCIW, x6 is TCLW, and x7 is sulfate AOD.
Figure 12 shows scatterplots and monthly distributions of CG lightning densities from multiple linear regression and observations in the plateau and basin regions. The scatterplots show that the modeled lightning density tends to be lower than the observed lightning density. The correlation in the basin region (R2=0.66) is higher than that in the plateau region (R2=0.51), but both are lower than the correlation reported by Stolz et al. (2017). Note that Stolz et al. (2017) examined total lightning on a global scale, whereas this study focuses on CG lightning formed over a region with complex terrain. The monthly distributions of observed and modeled CG lightning densities in the plateau and basin regions show that multiple linear regression can reproduce the seasonal variations in lightning activity well. Overall, the best agreement in both regions is seen in summer. The best agreements in the plateau and basin regions are seen in August and July, respectively.
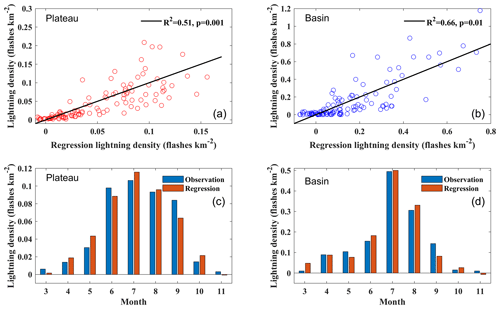
Figure 12Scatterplots of observed CG lightning densities as a function of lightning densities from multiple linear regression in the plateau and basin regions (a, b) and their monthly distributions (c, d).
To further discuss the main impact factors that contribute to lightning, we use the stepwise regression method to select the top three impact factors. The stepwise regression equations based on the top three impact factors are as follows:
for the plateau region,
and for the basin region,
where Y is the CG lightning density, x1 is CAPE, x2 is RH, x4 is CBH, x5 is TCIW, and x7 is sulfate AOD. The top three factors contributing to lightning in the plateau region are CAPE, RH, and CBH, and the top three factors contributing to lightning in the basin region are CAPE, TCIW, and AOD, suggesting that aerosols have a more prominent effect on lightning in the basin region.
Figure 13 shows scatterplots and monthly distributions of CG lightning densities from stepwise regression and observations in the plateau and basin regions. As seen in Fig. 12, the modeled lightning density tends to be lower than the observed lightning density, with R2 values of 0.51 and 0.66 in the plateau and basin regions, respectively. This also suggests that lightning activity can be reasonably modeled as long as factors that contribute significantly to lightning, such as CAPE, are properly determined. The monthly distributions of lightning densities modeled by stepwise regression agree with observations from March to October reasonably well.
In this study, we investigated the influences of aerosol, thermodynamic, and cloud-related factors on CG lightning activity in the plateau and basin regions of Sichuan Province, a part of China with complex terrain. Data used to discuss the dependence of the effect of aerosols on CG lightning on thermodynamic and cloud-related conditions included the CG lightning density, sulfate AOD, CAPE, RH, SHEAR, CBH, TCLW, and TCIW from 2005 to 2017.
CG lightning activity over the basin region was much more vigorous than that over the plateau region, mainly because the CAPE in the basin area was significantly larger than that in the plateau region (Qie et al., 2003). AODs in the basin region were also significantly higher than those in the plateau region, mainly due to the large amounts of anthropogenic air pollutant emissions and the mountainous terrain around the basin area that is not conducive to the dispersion of air pollutants. CG lightning activity was positively correlated with AOD in the plateau region, but it was negatively correlated with AOD in the basin region. The correlation between sulfate AOD and lightning was stronger than that between total AOD and lightning, and as sulfate AOD accounted for a high proportion of the total AOD, this study focused on the role of sulfate AOD. The lightning density over the plateau region increased exponentially with increasing AOD, whereas the lightning density over the basin region decreased exponentially with increasing AOD.
CAPE, RH, and TCIW were significantly positively correlated with lightning activity, whereas SHEAR was negatively correlated with lightning, suggesting that convective uplift and ice-phase particles are essential factors for lightning activity. CBH indirectly represents the warm-cloud thickness and is negatively correlated with TCLW. The increase in TCLW in the plateau region is beneficial to lightning activity, but this is not the case in the basin region, which may be related to the difference in warm-cloud depths between the two regions. In the plateau region, because of the compression effect of the plateau topography on clouds, warm clouds are very thin, and the high liquid water content is conducive to conveying more supercooled water to the freezing level, promoting the development of ice-phase clouds and lightning activity. In the basin region, higher liquid water contents mean robust warm-cloud processes, which are more conducive to the formation of warm rain than ice-phase processes, thereby inhibiting lightning activity. Partial correlation analyses indicate that CAPE, SHEAR, and TCIW are important factors controlling lightning activity, especially CAPE.
To reveal the joint effects of aerosol, thermodynamic, and cloud-related factors on CG lightning, AOD, CAPE, SHEAR, TCLW, and TCIW were selected for further analysis. In the plateau region, the aerosol loading is relatively low, stimulating lightning activity through the microphysical effect. An increase in aerosol loading reduces the size of cloud droplets, generating more but smaller cloud droplets, thereby reducing the collision–coalescence efficiency and inhibiting the warm-rain process. An increase in the liquid water content of a cloud is conducive to the development of the ice-phase process, which releases more latent heat and further stimulates convection. The increased convection and the increase in ice particles lead to more intense lightning activity. In the basin region, the aerosol loading is very high, which inhibits lightning activity through the radiative effect. High concentrations of aerosols reduce the solar radiation reaching the surface through absorption and scattering and decrease the convective energy from the ground to the lower atmosphere. The weakening of the convective uplift is not conducive to the transportation of liquid water above the freezing level and inhibits the development of the ice-phase process. Thus, the weakening of convection and the ice-phase process inhibits the intensity of lightning activity. The correlation between RLm and AOD and the correlation between RLr and AOD further the idea that aerosols over the plateau region affect the hydrometeor content in the atmosphere through the microphysical effect, whereas aerosols over the basin region mainly affect convective energy through the radiative effect, both of which impact lightning activity differently.
Our current study is limited to discussing the effect of aerosols on CG lightning density. Previous studies have suggested that aerosols affect the intensity and polarity of CG lightning (Lyons et al., 1998; Naccarato et al., 2003; Pawar et al., 2017). Future studies involving observational data analyses and numerical simulations will investigate the mechanism by which aerosols affect the CG lightning polarity by modulating the charge structure.
The CG lightning data can be obtained by contacting the first author (zpg@cuit.edu.cn). MERRA-2 aerosol data can be download from https://doi.org/10.5067/FH9A0MLJPC7N (GMAO, 2015), the ERA5 data can be downloaded from https://doi.org/10.24381/cds.f17050d7 (Copernicus Climate Change Service, 2017), the MISR aerosol data can be downloaded from https://doi.org/10.5067/Terra/MISR/MIL3MAEN_L3.004 (lNASA/LARC/SD/ASDC, 2008), and the CLARA-A2 data are available from https://doi.org/10.5676/EUM_SAF_CM/CLARA_AVHRR/V002 (Karlsson et al., 2017b).
The supplement related to this article is available online at: https://doi.org/10.5194/acp-20-13379-2020-supplement.
PZ and ZL designed the research ideas for this study. PZ carried the study out and prepared the paper. HX, YoZ, FW, XJ, and YuZ provided the analysis ideas for the meteorological and cloud-related parameters. MCC edited the paper.
The authors declare that they have no conflict of interest.
This research was jointly supported by the National Natural Science Foundation of China (grant nos. 41905126, 41875169, and 41705120), the National Key Research and Development Project (grant no. 2018YFC1505702), and the Key Laboratory for Cloud Physics of China Meteorological Administration LCP/CMA (grant no. 2017Z016). Pengguo Zhao acknowledges support from the China Scholarship Council (grant no. 201808515075).
This research has been supported by the National Natural Science Foundation of China (grant nos. 41905126, 41875169, and 41705120), the National Key Research and Development Project (grant no. 2018YFC1505702), the Key Laboratory for Cloud Physics of China Meteorological Administration LCP/CMA (grant no. 2017Z016), and the China Scholarship Council (grant no. 201808515075).
This paper was edited by Aijun Ding and reviewed by two anonymous referees.
Allen, D. J. and Pickering, K. E.: Evaluating lightning flash rate parameterizations for use in a global chemical transport model, J. Geophys. Res.-Atmos., 107, 4711, https://doi.org/10.1029/2002JD002066, 2002.
Altaratz, O., Koren, I., Yair, Y., and Price, C.: Lightning response to smoke from Amazonian fires, Geophys. Res. Lett., 37, L07801, https://doi.org/10.1029/2010GL042679, 2010.
Altaratz, O., Kucienska, B., Kostinski, A., Raga, G. B., and Koren, I.: Global association of aerosol with flash density of intense lightning, Environ. Res. Lett., 12, 114037, https://doi.org/10.1088/1748-9326/aa922b, 2017.
Bang, S. D. and Zipser E. J.: Seeking reasons for the differences in size spectra of electrified storms over land and ocean, J. Geophys. Res.-Atmos., 121, 9048–9068, https://doi.org/10.1002/2016JD025150, 2016.
Buchard, V., Randles, C. A., Da Silva, A. M., Darmenov, A., Colarco, P. R., Govindaraju, R., Ferrare, R., Hair, J., Beyersdorf, A. J., Ziemba, L. D., and Yu, H.: The MERRA-2 aerosol reanalysis, 1980 onward. Part II: Evaluation and case studies, J. Climate, 30, 6851–6872, https://doi.org/10.1175/JCLI-D-16-0613.1, 2017.
Carey, L. D. and Buffalo, K. M.: Environmental control of cloud-to-ground lightning polarity in severe storms, Mon. Weather Rev., 135, 1327–1353, https://doi.org/10.1175/MWR3361.1, 2007.
Carrió, G. G., and Cotton, W. R.: On the buffering of CCN impacts on wintertime orographic clouds: An idealized examination, Atmos. Res., 137, 136–144, https://doi.org/10.1016/j.atmosres.2013.09.011, 2014.
China Meteorological Administration, 2009: China Lightning Monitoring Reports, China Meteorological Press, Beijing, China, 142 pp., 2008 (in Chinese).
Copernicus Climate Change Service (C3S): ERA5: Fifth generation of ECMWF atmospheric reanalyses of the global climate, Copernicus Climate Change Service Climate Data Store (CDS), https://doi.org/10.24381/cds.f17050d7, 2017.
Cummins, K. L. and Murphy, M. J.: An overview of lightning locating systems: History, techniques, and data uses, with an in-depth look at the U.S. NLDN. IEEE Trans. Electromagn. Compat., 51, 499–518, https://doi.org/10.1109/TEMC.2009.2023450, 2009.
Cummins, K. L., Murphy, M. J., Bardo, E. A., Hiscox, W. L., Pyle, R. B., and Pifer, A. E.: A combined TOA/MDF technology upgrade of the U.S. National Lightning Detection Network, J. Geophys. Res.-Atmos., 103, 9035–9044, https://doi.org/10.1029/98JD00153, 1998.
Dafis, S., Fierro, A., Giannaros, T. M., Kotroni, V., Lagouvardos, K., and Mansell, E.: Performance evaluation of an explicit lightning forecasting system, J. Geophys. Res.-Atmos., 123, 5130–5148, https://doi.org/10.1029/2017JD027930, 2018.
Davies-Jones, R.: Linear and nonlinear propagation of supercell storms, J. Atmos. Sci., 59, 3178–3205, https://doi.org/10.1175/1520-0469(2003)059<3178:LANPOS>2.0.CO;2, 2002.
Dee, D. P., Uppala, S. M, Simmons, A. J., Berrisford, P., Poli, P., Kobayashi, S., Andrae, U., Balmaseda, M. A., Balsamo, G., Bauer, P., Bechtold, P., Beljaars, A. C. M., van de Berg, L., Bidlot, J., Bormann, A., Delsol, C., Dragani, R., Fuentes, M., Geer, A. J., Haimberger, L., Healy S. B., Hersbach, H., Hólm, E. V., Isaksen, L., Kållberg, P., Köhler, M., Matricardi, M., McNally, A. P., Monge‐Sanz, B. M., Morcrette, J. J., Park, B. K., Peubey, C., de Rosnay, P., Tavolato, C., Thépaut, J. N., and Vitart, F.: The ERA-Interim reanalysis: Configuration and performance of the data assimilation system, Q. J. R. Meteorol. Soc., 137, 553–597, https://doi.org/10.1002/qj.828, 2011.
Fan, J., Yuan, T., Comstock, J. M., Ghan, S., Khain, A., Leung, L. R., Li, Z., Martins, V. J., and Ovchinnikov, M.: Dominant role by vertical wind shear in regulating aerosol effects on deep convective clouds, J. Geophys. Res.-Atmos., 114, D22, https://doi.org/10.1029/2009JD012352, 2009.
Fan, J., Rosenfeld, D., Yang, Y., Zhao, C., Leung, L. R., and Li, Z.: Substantial contribution of anthropogenic air pollution to catastrophic floods in Southwest China, Geophys. Res. Lett., 42, 6066–6075, https://doi.org/10.1002/2015GL064479, 2015.
Fan, J., Rosenfeld, D., Zhang, Y., Giangrande, S., Li, Z., Machado, L., et al. Substantial convection and precipitation enhancements by ultrafine aerosol particles. Science, 359, 411–418, https://doi.org/10.1126/science.aan8461, 2018.
Freychet, N., Tett, S. F. B., Yan, Z., and Li, Z.: Underestimated change of wet-bulb temperatures over East and South China, Geophys. Res. Lett., 47, e2019GL086140, https://doi.org/10.1029/2019GL086140, 2020.
Fuchs, B. R., Rutledge, S. A., Bruning, E. C., Pierce, J. R., Kodros, J. K., Lang, T. J., MacGorman, D. R., Krehbiel, P. R., and Rison, W.: Environmental controls on storm intensity and charge structure in multiple regions of the continental United States, J. Geophys. Res.-Atmos., 120, 6575–6596, https://doi.org/10.1002/2015JD023271, 2015.
Global Modeling and Assimilation Office (GMAO): MERRA-2 tavgM_2d_aer_Nx: 2d,Monthly mean,Time-averaged,Single-Level,Assimilation,Aerosol Diagnostics V5.12.4, Greenbelt, MD, USA, Goddard Earth Sciences Data and Information Services Center (GES DISC), https://doi.org/10.5067/FH9A0MLJPC7N, 2015.
Guo, J., Deng, M., Lee, S.S., Wang, F., Li, Z., Zhai, P., Liu, H., Lv, W., Yao, W., and Li, X.: Delaying precipitation and lightning by air pollution over the Pearl River Delta. Part I: Observational analyses, J. Geophys. Res.-Atmos., 121, 6472–6488, https://doi.org/10.1002/2015JD023257, 2016.
Hoffmann, L., Günther, G., Li, D., Stein, O., Wu, X., Griessbach, S., Heng, Y., Konopka, P., Müller, R., Vogel, B., and Wright, J. S.: From ERA-Interim to ERA5: the considerable impact of ECMWF's next-generation reanalysis on Lagrangian transport simulations, Atmos. Chem. Phys., 19, 3097–3124, https://doi.org/10.5194/acp-19-3097-2019, 2019.
Huang, J., Zhang, C., and Prospero, J. M.: Large-scale effect of aerosols on precipitation in the West African Monsoon region, Q. J. R. Meteorol. Soc., 135, 581–594, https://doi.org/10.1002/qj.391, 2009.
Jiang, J. H., Su, H., Huang, L., Wang, Y., Massie, S., Zhao, B., Omar A, and Wang, Z.: Contrasting effects on deep convective clouds by different types of aerosols, Nat. Commun., 9, 1–7, https://doi.org/10.1038/s41467-018-06280-4, 2018.
Kar, S. K. and Liou, Y. A.: Enhancement of cloud-to-ground lightning activity over Taipei, Taiwan in relation to urbanization, Atmos. Res., 147–148, 111–120, https://doi.org/10.1016/j.atmosres.2014.05.017, 2014.
Kar, S. K., Liou, Y. A., and Ha, K. J.: Aerosol effects on the enhancement of cloud-to-ground lightning over major urban areas of South Korea, Atmos. Res., 92, 80–87, https://doi.org/10.1016/j.atmosres.2008.09.004, 2009.
Karlsson, K.-G. and Håkansson, N.: Characterization of AVHRR global cloud detection sensitivity based on CALIPSO-CALIOP cloud optical thickness information: demonstration of results based on the CM SAF CLARA-A2 climate data record, Atmos. Meas. Tech., 11, 633–649, https://doi.org/10.5194/amt-11-633-2018, 2018.
Karlsson, K.-G., Anttila, K., Trentmann, J., Stengel, M., Fokke Meirink, J., Devasthale, A., Hanschmann, T., Kothe, S., Jääskeläinen, E., Sedlar, J., Benas, N., van Zadelhoff, G.-J., Schlundt, C., Stein, D., Finkensieper, S., Håkansson, N., and Hollmann, R.: CLARA-A2: the second edition of the CM SAF cloud and radiation data record from 34 years of global AVHRR data, Atmos. Chem. Phys., 17, 5809–5828, https://doi.org/10.5194/acp-17-5809-2017, 2017a.
Karlsson, K.-G., Anttila, K., Trentmann, J., Stengel, M., Meirink, J. F., Devasthale, A., Hanschmann, T., Kothe, S., Jääskeläinen, E., Sedlar, J., Benas, N., van Zadelhoff, G.-J., Schlundt, C., Stein, D., Finkensieper, S., Håkansson, N., Hollmann, R., Fuchs, P., and Werscheck, M.: CLARA-A2: CM SAF cLoud, Albedo and surface RAdiation dataset from AVHRR data – Edition 2, Satellite Application Facility on Climate Monitoring, https://doi.org/10.5676/EUM_SAF_CM/CLARA_AVHRR/V002, 2017b.
Khain, A., Cohen, N., Lynn, B., and Pokrovsky, A.: Possible aerosol effects on lightning activity and structure of hurricanes, J. Atmos. Sci., 65, 3652–3667, https://doi.org/10.1175/2008JAS2678.1, 2008.
Koren, I., Martins, J. V., Remer, L. A., and Afargan, H.: Smoke invigoration versus inhibition of clouds over the Amazon, Science, 321, 946–949, https://doi.org/10.1126/science.1159185, 2008.
Koren, I., Altaratz, O., Remer, L. A., Feingold, G., Martins, J. V., and Heiblum, R. H.: Aerosol-induced intensification of rain from the tropics to the mid-latitudes, Nat. Geosci., 5, 118–122, https://doi.org/10.1038/ngeo1364, 2012.
Koren, I., Dagan, G., and Altaratz, O.: From aerosol-limited to invigoration of warm convective clouds, Science, 344, 1143–1146, 2014.
Lee, S. S., Guo, J., and Li, Z.: Delaying precipitation by air pollutionover the Pearl River Delta: 2. Model simulations, J. Geophys. Res.-Atmos., 121, 11739–11760, https://doi.org/10.1002/2015JD024362, 2016.
Lee, S., Hwang, S. O., Kim, J., and Ahn, M. H.: Characteristics of cloud occurrence using ceilometer measurements and its relationship to precipitation over Seoul, Atmos. Res., 201, 46–57, https://doi.org/10.1016/j.atmosres.2017.10.010, 2018.
Lei, Y., Letu, H., Shang, H., and Shi, J.: Cloud cover over the Tibetan Plateau and eastern China: a comparison of ERA5 and ERA-Interim with satellite observations, Clim. Dynam., 54, 2941–2957, https://doi.org/10.1007/s00382-020-05149-x, 2020.
Li, X., Guo, X., and Fu, D.: TRMM-retrieved cloud structure and evolution of MCSs over the northern South China Sea and impacts of CAPE and vertical wind shear, Adv. Atmos. Sci., 30, 77–88, https://doi.org/10.1007/s00376-012-2055-2, 2013.
Li, X., Pan, Y., and Mo, Z.: Joint effects of several factors on cloud-to-ground lightning and rainfall in Nanning (China), Atmos. Res., 212, 23–32, https://doi.org/10.1016/j.atmosres.2018.05.002, 2018.
Li, Z., Niu, F., Fan, J., Liu, Y., Rosenfeld, D., and Ding, Y.: Long-term impacts of aerosols on the vertical development of clouds and precipitation, Nat. Geosci., 4, 888–894, https://doi.org/10.1038/ngeo1313, 2011.
Li, Z., Rosenfeld, D., and Fan, J.: Aerosols and their impact on radiation, clouds, precipitation, and severe weather events, Oxford Research Encyclopedias, PNNL-SA-124900, Oxford, England, Oxford University Press, available at: https://oxfordre.com/environmentalscience/view/10.1093/acrefore/9780199389414.001.0001/acrefore-9780199389414-e-126 (last access: 30 March 2020), 2017.
Li, Z., Wang, Y., Guo, J., Zhao, C., Cribb, M. C., Dong, X., Fan, J., Gong, D., Huang, J., Jiang, M., and Jiang, Y.: East Asian Study of Tropospheric Aerosols and their Impact on Regional Clouds, Precipitation, and Climate (EAST-AIRCPC), J. Geophys. Res.-Atmos., 124, 13026–13054, https://doi.org/10.1029/2019JD030758, 2019.
Liu, H., Guo, J., Koren, I., Altaratz, O., Dagan, G., Wang, Y., Jiang, J. H., Zhai, P., and Yung, Y. L.: Non-monotonic aerosol effect on precipitation in convective clouds over tropical oceans, Sci. Rep., 9, 1–7, https://doi.org/10.1038/s41598-019-44284-2, 2019.
Lyons, W. A., Nelson, T. E., Williams, E. R., Cramer, J. A., and Turner, T. R.: Enhanced positive cloud-to-ground lightning in thunderstorms ingesting smoke from fires, Science, 282, 77–80, https://doi.org/10.1126/science.282.5386.77, 1998.
MacGorman, D. R., Straka, J. M., and Ziegler, C. L.: A lightning parameterization for numerical cloud models, J. Appl. Meteorol., 40, 459–478, https://doi.org/10.1175/1520-0450(2001)040<0459:ALPFNC>2.0.CO;2, 2001.
Mansell, E. R. and Ziegler, C. L.: Aerosol Effects on Simulated Storm Electrification and Precipitation in a Two-Moment Bulk Microphysics Model, J. Atmos. Sci., 70, 2032–2050, https://doi.org/10.1175/JAS-D-12-0264.1, 2013.
Mansell, E. R., MacGorman, D. R., Ziegler, C. L., and Straka, J. M.: Charge structure and lightning sensitivity in a simulated multicell thunderstorm, J. Geophys. Res.-Atmos., 110, 1545–1555, https://doi.org/10.1029/2004JD005287, 2005.
Minzner, R. A.: The 1976 standard atmosphere and its relationship to earlier standards, Rev. Geophys., 15, 375–384, https://doi.org/10.1029/RG015i003p00375, 1977.
Naccarato, K. P., Pinto Jr., O., and Pinto, I. R. C. A.: Evidence of thermal and aerosol effects on the cloud-to-ground lightning density and polarity over large urban areas of Southeastern Brazil, Geophys. Res. Lett., 30, 1674, https://doi.org/10.1029/2003GL017496, 2003.
NASA/LARC/SD/ASDC: MISR Level 3 Component Global Aerosol product in netCDF format covering a month V004, https://doi.org/10.5067/Terra/MISR/MIL3MAEN_L3.004, 2008.
Ning, G., Wang, S., Ma, M., Ni, C., Shang, Z., Wang, J., and Li, J.: Characteristics of air pollution in different zones of Sichuan Basin, China, Sci. Total Environ., 612, 975–984, https://doi.org/10.1016/j.scitotenv.2017.08.205, 2018a.
Ning, G., Wang, S., Yim, S. H. L., Li, J., Hu, Y., Shang, Z., Wang, J., and Wang, J.: Impact of low-pressure systems on winter heavy air pollution in the northwest Sichuan Basin, China, Atmos. Chem. Phys., 18, 13601–13615, https://doi.org/10.5194/acp-18-13601-2018, 2018b.
Ning, G., Yim, S.H.L., Wang, S., Duan, B., Nie, C., Yang, X., Wang, J., and Shang, K.: Synergistic effects of synoptic weather patterns and topography on air quality: a case of the Sichuan Basin of China, Clim. Dynam., 53, 6729–6744, https://doi.org/10.1007/s00382-019-04954-3, 2019.
Oreopoulos, L., Cho, N., and Lee, D.: A global survey of apparent aerosol-cloud interaction signals, J. Geophys. Res., 125, e2019JD031287, https://doi.org/10.1029/2019JD031287, 2020.
Orville, R. E., Huffines, G. R., Burrows, W. R., and Cummins, K. L.: The North American lightning detection network (NALDN)-Analysis of flash data: 2001–09, Mon. Weather Rev., 139, 1305–1322, https://doi.org/10.1175/2010MWR3452.1, 2011.
Pawar, S. D., Gopalakrishnan, V., Murugavel, P., Veremey, N. E., and Sinkevich, A. A.: Possible role of aerosols in the charge structure of isolated thunderstorms, Atmos. Res., 183, 331–340, https://doi.org/10.1016/j.atmosres.2016.09.016, 2017.
Pinto, I. R. C. A., Pinto Jr., O., Gomes, M. A. S. S., and Ferreira, N. J.: Urban effect on the characteristics of cloud-to-ground lightning over Belo Horizonte-Brazil, Ann. Geophys., 22, 697–700, 2004.
Price, C. G.: Lightning Applications in Weather and Climate Research, Surv. Geophys., 34, 755–767, https://doi.org/10.1007/s10712-012-9218-7, 2013.
Proestakis, E., Kazadzis, S., Lagouvardos, K., Kotroni, V., and Kazantzidis, A.: Lightning activity and aerosols in the Mediterranean region, Atmos. Res., 170, 66–75, https://doi.org/10.1016/j.atmosres.2015.11.010, 2016.
Qian, Y., Gong, D., Fan, J., Leung, L. R., Bennartz, R., Chen, D., and Wang, W.: Heavy pollution suppresses light rain in China: Observations and modeling, J. Geophys. Res.-Atmos., 114, D00K02, https://doi.org/10.1029/2008JD011575, 2009.
Qie, X. and Zhang, Y.: A Review of Atmospheric Electricity Research in China from 2011 to 2018, Adv. Atmos. Sci., 36, 994–1014, https://doi.org/10.1007/s00376-019-8195-x, 2019.
Qie, X., Toumi, R., and Zhou, Y. J.: Lightning activity on the central Tibetan Plateau and its response to convective available potential energy, Chin. Sci. Bull., 48, 296–299, https://doi.org/10.1007/BF03183302, 2003.
Ramos, A. M., Ramos, R., Sousa, P., Trigo, R. M., Janeira, M., and Prior, V.: Cloud to ground lightning activity over Portugal and its association with circulation weather types, Atmos. Res., 101, 84–101, https://doi.org/10.1016/j.atmosres.2011.01.014, 2011.
Randles, C. A., Da Silva, A. M., Buchard, V., Colarco, P. R., Darmenov, A., Govindaraju, R., Smirnov, A., Holben, B., Ferrare, R., Hair, J., and Shinozuka, Y.: The MERRA-2 aerosol reanalysis, 1980 onward. Part I: System description and data assimilation evaluation, J. Climate, 30, 6823–6850, https://doi.org/10.1175/JCLI-D-16-0609.1, 2017.
Romps, D. M., Seeley, J. T., Vollaro, D., and Molinari, J.: Projected increase in lightning strikes in the United States due to global warming, Science, 346, 851–854, https://doi.org/10.1126/science.1259100, 2014.
Romps, D. M., Charn, A. B., Holzworth, R. H., Lawrence, W. E., Molinari, J., and Vollaro, D.: CAPE times P explains lightning over land but not the land-ocean contrast, Geophys. Res. Lett., 45, 12623–12630, https://doi.org/10.1029/2018GL080267, 2018.
Rosenfeld, D., Dai, J., Yu, X., Yao, Z., Xu, X., Yang, X., and Du, C.: Inverse relations between amounts of air pollution and orographic precipitation, Science, 315, 1396–1398, https://doi.org/10.1126/science.1137949, 2007.
Rosenfeld, D., Lohmann, U., Raga, G. B., O'Dowd, C. D., Kulmala, M., Fuzzi, S., Reissell, A., and Andreae, M. O.: Flood or Drought: How Do Aerosols Affect Precipitation, Science, 321, 1309–1313, https://doi.org/10.1126/science.1160606, 2008.
Saunders, C.: Charge separation mechanisms in clouds, Space Sci. Rev., 137, 335–353, https://doi.org/10.1007/s11214-008-9345-0, 2008.
Saunders, C. P. R., Keith, W. D., and Mitzeva, R. P.: The effect of liquid water on thunderstorm charging, J. Geophys. Res.-Atmos., 96, 11007–11017, https://doi.org/10.1029/91JD00970, 1991.
Sherburn, K. D., Parker, M. D., King, J. R., and Lackmann, G. M.: Composite environments of severe and nonsevere high-shear, low-CAPE convective events, Weather Forecast., 31, 1899–1927, https://doi.org/10.1175/WAF-D-16-0086.1, 2016.
Shi, Z., Tan, Y., Tang, H., Sun, J., Yang, Y., Peng, L., and Guo, X.: Aerosol effect on the land-ocean contrast in thunderstorm electrification and lightning frequency, Atmos. Res., 164–165, 131–141, https://doi.org/10.1016/j.atmosres.2015.05.006, 2015.
Shou, Y., Lu, F., Liu, H., Cui, P., Shou, S., and Liu, J.: Satellite-based observational study of the Tibetan Plateau Vortex: Features of deep convective cloud tops, Adv. Atmos. Sci., 36, 189–205, https://doi.org/10.1007/s00376-018-8049-y, 2019.
Stallins, J. A., Carpenter, J., Bentley, M. L., Ashley, W. S., and Mulholland, J. A.: Weekend-weekday aerosols and geographic variability in cloud-to-ground lightning for the urban region of Atlanta, Georgia, USA, Reg. Environ. Change, 13, 137–151, https://doi.org/10.1007/s10113-012-0327-0, 2013.
Stolz, D. C., Rutledge, S. A., and Pierce, J. R.: Simultaneous influences of thermodynamics and aerosols on deep convection and lightning in the tropics, J. Geophys. Res.-Atmos., 120, 6207–6231, https://doi.org/10.1002/2014JD023033, 2015.
Stolz, D. C., Rutledge, S. A., Pierce, J. R., and van den Heever, S. C.: A global lightning parameterization based on statistical relationships among environmental factors, aerosols, and convective clouds in the TRMM climatology, J. Geophys. Res.-Atmos., 122, 7461–7492, https://doi.org/10.1002/2016JD026220, 2017.
Sun, L., Wei, J., Duan, D. H., Guo, Y. M., Yang, D. X., Jia, C., and Mi, X.: Impact of Land-Use and Land-Cover Change on urban air quality in representative cities of China, J. Atmos. Sol.-Terr. Phy., 142, 43–54, https://doi.org/10.1016/j.jastp.2016.02.022, 2016.
Sun, E., Che, H., Xu, X., Wang, Z., Lu, C., Gui, K., Zhao, H., Zheng, Y., Wang, Y., Wang, H., and Sun, T.: Variation in MERRA-2 aerosol optical depth over the Yangtze River Delta from 1980 to 2016, Theor. Appl. Climatol., 136, 363–375, https://doi.org/10.1007/s00704-018-2490-9, 2019a.
Sun, E., Xu, X., Che, H., Tang, Z., Gui, K., An, L., Lu, C., and Shi. G.: Variation in MERRA-2 aerosol optical depth and absorption aerosol optical depth over China from 1980 to 2017, J. Atmos. Sol.-Terr. Phy., 186, 8–19, https://doi.org/10.1016/j.jastp.2019.01.019, 2019b.
Tan, Y., Peng, L., Shi, Z., and Chen, H.: Lightning flash density in relation to aerosol over Nanjing (China), Atmos. Res., 174, 1–8, https://doi.org/10.1016/j.atmosres.2016.01.009, 2016.
Thompson, R. L., Mead, C. M., and Edwards, R.: Effective storm-relative helicity and bulk shear in supercell thunderstorm environments, Weather Forecast., 22, 102–115, https://doi.org/10.1175/WAF969.1, 2007.
Thornton, J. A., Virts, K. S., Holzworth, R. H., and Mitchell, T. P.: Lightning enhancement over major oceanic shipping lanes, Geophys. Res. Lett., 44, 9102–9111, https://doi.org/10.1002/2017GL074982, 2017.
Tinmaker, M. I. R., Ghude, S. D., and Chate, D. M.: Land-sea contrasts for climatic lightning activity over Indian region, Theor. Appl. Climatol., 138, 931–940, https://doi.org/10.1007/s00704-019-02862-4, 2019.
Tippett, M. K. and Koshak, W. J.: A baseline for the predictability of U.S. cloud-to-ground lightning, Geophys. Res. Lett., 45, 10719–10728, https://doi.org/10.1029/2018GL079750, 2018.
Tippett, M. K., Lepore, C., Koshak, W. J., Chronis, T., and Vant-Hull, B.: Performance of a simple reanalysis proxy for US cloud-to-ground lightning, Int. J. Climatol., 39, 3932–3946, https://doi.org/10.1002/joc.6049, 2019.
Wall, C., Zipser, E. J., and Liu, C.: An investigation of the aerosol indirect effect on convective intensity using satellite observations, J. Atmos. Sci., 71, 430–447, https://doi.org/10.1175/JAS-D-13-0158.1, 2014.
Wang, Y., Wan, Q., Meng, W., Liao, F., Tan, H., and Zhang, R.: Long-term impacts of aerosols on precipitation and lightning over the Pearl River Delta megacity area in China, Atmos. Chem. Phys., 11, 12421–12436, https://doi.org/10.5194/acp-11-12421-2011, 2011.
Wang, Y., Khalizov, A., Levy, M., and Zhang, R.: New Directions: Light absorbing aerosols and their atmospheric impacts, Atmos. Environ., 81, 713–715, https://doi.org/10.1016/j.atmosenv.2013.09.034, 2013.
Wang, Q., Li, Z., Guo, J., Zhao, C., and Cribb, M.: The climate impact of aerosols on the lightning flash rate: is it detectable from long-term measurements?, Atmos. Chem. Phys., 18, 12797–12816, https://doi.org/10.5194/acp-18-12797-2018, 2018.
Wei, J., Huang, W., Li, Z., Xue, W., Peng, Y., Sun, L., and Cribb, M.: Estimating 1-km-resolution PM2.5 concentrations across China using the space-time random forest approach, Remote Sens. Environ., 231, 111221, https://doi.org/10.1016/j.rse.2019.111221, 2019a.
Wei, J., Li, Z., Guo, J., Sun, L., Huang, W., Xue, W., Fan, T, and Cribb, M. Satellite-derived 1-km-resolution PM1 concentrations from 2014 to 2018 across China, Environ. Sci. Technol., 53, 13265–13274, https://doi.org/10.1021/acs.est.9b03258, 2019b.
Wei, J., Peng, Y., Mahmood, R., Sun, L., and Guo, J.: Intercomparison in spatial distributions and temporal trends derived from multi-source satellite aerosol products, Atmos. Chem. Phys., 19, 7183–7207, https://doi.org/10.5194/acp-19-7183-2019, 2019c.
Westcott, N. E.: Summertime cloud-to-ground lightning activity around major Midwestern urban areas, J. Appl. Meteorol., 34, 1633–1642, https://doi.org/10.1175/1520-0450-34.7.1633, 1995.
Williams, E. R.: Lightning and climate: A review, Atmos. Res., 76, 272–287, https://doi.org/10.1016/j.atmosres.2004.11.014, 2005.
Williams, E. and Stanfill, S.: The physical origin of the land-ocean contrast in lightning activity, C. R. Phys., 3, 1277–1292, https://doi.org/10.1016/S1631-0705(02)01407-X, 2002.
Williams, E. R., Chan, T., and Boccippio, D.: Islands as miniature continents: another look at the land ocean lightning contrast, J. Geophys. Res.-Atmos., 109, D16206, https://doi.org/10.1029/2003JD003833, 2004.
Williams, E. R., Mushtak, V., Rosenfeld, D., Goodman, S., and Boccippio, D.: Thermodynamic conditions favorable to superlative thunderstorm updraft, mixed phase microphysics and lightning flash rate, Atmos. Res., 76, 288–306, https://doi.org/10.1016/j.atmosres.2004.11.009, 2005.
Wong, J., Barth, M. C., and Noone, D.: Evaluating a lightning parameterization based on cloud-top height for mesoscale numerical model simulations, Geosci. Model Dev., 6, 429–443, https://doi.org/10.5194/gmd-6-429-2013, 2013.
Xia, R., Zhang, D. L., and Wang, B.: A 6-yr cloud-to-ground lightning climatology and its relationship to rainfall over central and eastern China, J. Appl. Meteorol. Clim., 54, 2443–2460, https://doi.org/10.1175/JAMC-D-15-0029.1, 2015.
Yair, Y.: Lightning hazards to human societies in a changing climate, Environ. Res. Lett., 13, 123002, https://doi.org/10.1088/1748-9326/aaea86, 2018.
Yair, Y., Lynn, B., Price, C., Kotroni, V., Lagouvardos, K., Morin, E., Mugnai, A., and Llasat, M. D. C.: Predicting the potential for lightning activity in Mediterranean storms based on the Weather Research and Forecasting (WRF) model dynamic and microphysical fields, J. Geophys. Res.-Atmos., 115, D04205, https://doi.org/10.1029/2008JD010868, 2010.
Yang, X., and Li, Z.: Increases in thunderstorm activity and relationships with air pollution in southeast China, J. Geophys. Res.-Atmos., 119, 1835–1844, https://doi.org/10.1002/2013JD021224, 2014.
Yang, X., Yao, Z., Li, Z., and Fan, T.: Heavy air pollution suppresses summer thunderstorms in central China, J. Atmos. Sol.-Terr. Phy., 95, 28–40, https://doi.org/10.1016/j.jastp.2012.12.023, 2013.
Yang, X., Sun, J., and Li, W.: An analysis of cloud-to-ground lightning in China during 2010–13, Weather Forecast., 30, 1537–1550, https://doi.org/10.1175/WAF-D-14-00132.1, 2015.
Yang, X., Li, Z., Liu, L., Zhou, L., Cribb, M., and Zhang, F.: Distinct weekly cycles of thunderstorms and a potential connection with aerosol type in China, Geophys. Res. Lett., 43, 8760–8768, https://doi.org/10.1002/2016GL070375, 2016.
Yu, R., Xu, Y., Zhou, T., and Li, J.: Relation between rainfall duration and diurnal variation in the warm season precipitation over central eastern China, Geophys. Res. Lett., 34, L13703, https://doi.org/10.1029/2006GL028129, 2007.
Yuan, T., Remer, L. A., Pickering, K. E., and Yun, H.: Observational evidence of aerosol enhancement of lightning activity and convective invigoration, Geophys. Res. Lett., 38, L04701, https://doi.org/10.1029/2010GL046052, 2011.
Zhang, X. Y., Wang, Y. Q., Niu, T., Zhang, X. C., Gong, S. L., Zhang, Y. M., and Sun, J. Y.: Atmospheric aerosol compositions in China: spatial/temporal variability, chemical signature, regional haze distribution and comparisons with global aerosols, Atmos. Chem. Phys., 12, 779–799, https://doi.org/10.5194/acp-12-779-2012, 2012.
Zhang, Y., Sun, J., and Fu, S.: Impacts of diurnal variation of mountain-plain solenoid circulations on precipitation and vortices east of the Tibetan Plateau during the mei-yu season, Adv. Atmos. Sci., 31, 139–153, https://doi.org/10.1007/s00376-013-2052-0, 2014.
Zhang, Y., Cai, C., Chen, B., and Dai, W.: Consistency evaluation of precipitable water vapor derived from ERA5, ERA-Interim, GNSS, and radiosondes over China, Radio Sci., 54, 561–571, https://doi.org/10.1029/2018RS006789, 2019.
Zhao, B., Wang, Y., Gu, Y., Liou, K. N., Jiang, J. H., Fan, J., Liu, X., Lei, H., and Yung, Y. L.: Ice nucleation by aerosols from anthropogenic pollution, Nat. Geosci., 12, 602–607, https://doi.org/10.1038/s41561-019-0389-4, 2019.
Zhao, C., Tie, X., and Lin, Y.: A possible positive feedback of reduction of precipitation and increase in aerosols over eastern central China, Geophys. Res. Lett., 33, L11814, https://doi.org/10.1029/2006GL025959, 2006.
Zhao, C., Lin, Y., Wu, F., Wang, Y., Li, Z., Rosenfeld, D., and Wang, Y.: Enlarging rainfall area of tropical cyclones by atmospheric aerosols, Geophys. Res. Lett., 45, 8604–8611, https://doi.org/10.1029/2018GL079427, 2018.
Zhao, P., Yin, Y., and Xiao, H.: The effects of aerosol on development of thunderstorm electrification: A numerical study, Atmos. Res., 153, 376–391, https://doi.org/10.1016/j.atmosres.2014.09.011, 2015.