the Creative Commons Attribution 4.0 License.
the Creative Commons Attribution 4.0 License.
Analysis of the distributions of hourly NO2 concentrations contributing to annual average NO2 concentrations across the European monitoring network between 2000 and 2014
Christopher S. Malley
Erika von Schneidemesser
Sarah Moller
Christine F. Braban
W. Kevin Hicks
Mathew R. Heal
Exposure to nitrogen dioxide (NO2) is associated with negative human health effects, both for short-term “peak” concentrations and from long-term exposure to a wider range of NO2 concentrations. For the latter, the European Union has established an air quality limit value of 40 µg m−3 as an annual average. However, factors such as proximity and strength of local emissions, atmospheric chemistry, and meteorological conditions mean that there is substantial variation in the hourly NO2 concentrations contributing to an annual average concentration. The aim of this analysis was to quantify the nature of this variation at thousands of monitoring sites across Europe through the calculation of a standard set of “chemical climatology” statistics. Specifically, at each monitoring site that satisfied data capture criteria for inclusion in this analysis, annual NO2 concentrations, as well as the percentage contribution from each month, hour of the day, and hourly NO2 concentrations divided into 5 µg m−3 bins were calculated.
Across Europe, 2010–2014 average annual NO2 concentrations (NO2AA) exceeded the annual NO2 limit value at 8 % of > 2500 monitoring sites. The application of this “chemical climatology” approach showed that sites with distinct monthly, hour of day, and hourly NO2 concentration bin contributions to NO2AA were not grouped into specific regions of Europe, furthermore, within relatively small geographic regions there were sites with similar NO2AA, but with differences in these contributions. Specifically, at sites with highest NO2AA, there were generally similar contributions from across the year, but there were also differences in the contribution of peak vs. moderate hourly NO2 concentrations to NO2AA, and from different hours across the day. Trends between 2000 and 2014 for 259 sites indicate that, in general, the contribution to NO2AA from winter months has increased, as has the contribution from the rush-hour periods of the day, while the contribution from peak hourly NO2 concentrations has decreased. The variety of monthly, hour of day and hourly NO2 concentration bin contributions to NO2AA, across cities, countries and regions of Europe indicate that within relatively small geographic areas different interactions between emissions, atmospheric chemistry and meteorology produce variation in NO2AA and the conditions that produce it. Therefore, measures implemented to reduce NO2AA in one location may not be as effective in others. The development of strategies to reduce NO2AA for an area should therefore consider (i) the variation in monthly, hour of day, and hourly NO2 concentration bin contributions to NO2AA within that area; and (ii) how specific mitigation actions will affect variability in hourly NO2 concentrations.
- Article
(10155 KB) - Full-text XML
-
Supplement
(5189 KB) - BibTeX
- EndNote
Nitrogen dioxide (NO2) is an air pollutant that is directly emitted and together with nitric oxide (NO) constitutes primary emitted nitrogen oxides (commonly termed NOx). NO2 is emitted from a range of sources, most importantly from road transport (with different vehicle types emitting different proportions of NOx as NO2), and is also formed in the atmosphere through the reaction between primary emitted NO and ozone (O3). Exposure to NO2 has been linked to negative human health effects, as summarised in the World Health Organization's (WHO) Review of the Evidence on the Health Aspects of Air Pollution (REVIHAAP, 2013). Alongside their conclusion of “consistent” epidemiological evidence for association between short-term NO2 exposure and negative respiratory effects, REVIHAAP (2013) also conclude that studies that have used multi-pollutant models to separate the association between long-term NO2 exposure and respiratory effects from those of other pollutants “provide support for an effect of NO2 independent from particle mass metrics”, and that this evidence was “suggestive of a causal relationship”. The United States Environmental Protection Agency (US EPA) similarly concluded that epidemiological and experimental evidence indicates that there is “likely to be a causal relationship between long-term NO2 exposure and respiratory effects” (US EPA, 2016). The United Kingdom Committee on the Medical Effects of Air Pollution (COMEAP) concluded that “although it is possible that, to some extent, NO2 acts as a marker of the effects of other traffic-related pollutants, the epidemiological and mechanistic evidence now suggests that it would be sensible to regard NO2 as causing some of the health impact found to be associated with it in epidemiological studies” (COMEAP, 2015). Recent analysis of large prospective cohorts in the United States and Canada also indicate significant associations between long-term NO2 exposure and mortality after controlling for exposure to PM2.5 and O3 (Crouse et al., 2015; Turner et al., 2016).
In the European Union (EU), the evidence of NO2 health effects has led to the establishment of air quality standards for the protection of human health. Limit values for NO2 are set at 200 µg m−3 for 1 h average concentrations (with 18 exceedances permitted per year), and 40 µg m−3 for annual average concentrations (European Council Directive 2008/50/EC, 2008). These limit values are in line with the WHO air quality guidelines (WHO, 2006). Hence a common basis for assessment of NO2 levels relevant to the long-term health impact is well established across EU Member States. However, despite the consistent “impact” metric, a common approach to assess the conditions producing this impact metric is currently lacking. For the protection of human health and vegetation, understanding these conditions is important in addition to assessment of the extent of the impact metric itself, as it informs how the magnitude of the impact metric arises, and therefore the options available for its mitigation.
Annual average NO2 concentrations in a location are determined by a combination of factors, including the following.
- i.
The emissions environment, such as the proximity and magnitude of major sources of NOx (NO + NO2) and the proportion of NOx emissions that are directly emitted as NO2 (AQEG, 2007; Beevers et al., 2012; Carslaw et al., 2011; Carslaw and Beevers, 2004; Grice et al., 2009; Vestreng et al., 2009). Across Europe, the major source of NOx emissions is road transport (39 % of NOx emissions in the EU28 Member States in 2014; EEA, 2016b), and recent analysis has suggested that road transport NOx emissions could be even higher when accounting for real-world emission factors (Anenberg et al., 2017). Other sources include energy production and distribution (20 %), commercial, institutional and households (14 %), and energy use in industry (13 %) (EEA, 2016b).
- ii.
Atmospheric chemistry, including the balance between secondary NO2 formation through the reaction between primary emitted NO and O3, and its photolysis back to NO and O3 as emissions are transported further from their source (Jenkin, 2014; Jenkin and Clemitshaw, 2000; Mavroidis and Chaloulakou, 2011). Other chemical processes which determine levels of NO2 at a particular location include the reaction of NO2 with the hydroxyl (OH) radical to form nitric acid (HNO3), which is a major chemical removal process for NO2 during daytime (Jenkin and Clemitshaw, 2000), and the combination of NO2 and peroxy radicals to form peroxy acetyl nitrates (PANs) which also removes NO2 close to emission sources. PAN species can also be transported long distances and act as a source of NO2 remote from major emission sources (Fischer et al., 2014).
- iii.
Atmospheric dispersion between source and receptor (AQEG, 2004; Donnelly et al., 2012). Meteorological conditions determine NO2 levels by controlling the extent to which emissions can disperse (AQEG, 2004). For example, lower boundary layer heights and temperature inversions during winter can prevent pollution, including NO2, from ventilating from the boundary layer, leading to higher wintertime NO2 concentrations in European cities. In contrast, in summer, daytime boundary layer heights are generally higher, and there is greater solar activity which reduces NO2 concentrations in some locations through NO2 photolysis (Henschel et al., 2016). Additionally, the built environment also determines the extent to which road transport NOx emissions (or from other sources) can escape the street canyon, or immediate environment within which they are emitted. At background monitoring sites in rural locations, longer range transport can also determine NO2 concentrations (Donnelly et al., 2012).
The combination of emission, chemistry, and meteorology can drive substantial variation in the distributions of hourly NO2 concentrations across the year and across the day that underpin any given annual average NO2 concentration. The aim of this work was to investigate the spatial and temporal variation in the patterns of hourly NO2 concentrations producing annual average NO2 concentrations across Europe, utilising data from thousands of European NO2 monitoring sites. At each site, a standard set of statistics were calculated to quantify the magnitude of an “impact” metric, i.e. annual average NO2 concentrations, as well as the contribution to annual average NO2 concentrations from each month of the year, hour of the day, and from hourly NO2 concentrations (hourly concentrations were binned to concentration ranges in this study to investigate the influence of relatively low, moderate, or high hourly NO2 concentrations in determining annual NO2 concentrations). This method of integrating analysis of an impact metric with the variation in atmospheric composition producing it has been outlined previously as a “chemical climatology” framework (Malley et al., 2014a), and applied to assess the conditions producing long-term health relevant PM impacts, and ozone health and vegetation impacts, at the two UK European Monitoring and Evaluation Programme (EMEP) monitoring sites (Malley et al., 2015, 2016). The application here of the chemical climatology approach to assessment of long-term health-relevant NO2 is the first application of this methodology to a regional monitoring network encompassing thousands of monitoring sites.
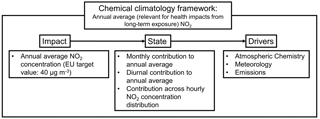
Figure 1Outline of chemical climatology elements used to evaluate the conditions producing “long-term health-relevant” NO2 concentrations across Europe.
Europe-wide NO2 concentrations have been examined previously. For example, Cyrys et al. (2012) investigated variation in annual NO2 concentrations across 36 European cities/regions to assess spatial differences in estimated NO2 exposure for use in epidemiological analysis of air pollution health impacts. Henschel et al. (2016) used monitoring data to assess trends (1999–2010) in NO2 concentrations in relation to the implementation of the Euro NOx emission standards in nine cities in seven countries. In addition, studies focussing on one city, country, or region of Europe have also been undertaken (AQEG, 2004; Braniš, 2009; Carslaw et al., 2011; Carugno et al., 2016; Chaloulakou et al., 2008; Clapp and Jenkin, 2001; Cuevas et al., 2014; Donnelly et al., 2012, 2011; Gilge et al., 2010; Jenkin, 2014; Lozano et al., 2009; Mavroidis and Chaloulakou, 2011). These studies differ in the methods for analysis of NO2 measurements, for example Henschel et al. (2016) select one traffic and urban background site to represent all traffic and urban background conditions in that city, while Cyrys et al. (2012) identify greater variation in annual NO2 concentrations within each city than between cities but did not assess variation in short-term NO2 concentration contributions to annual concentrations due to the two-week resolution of the measurements. This work builds on these previous studies by presenting a framework for the common characterisation of not only the magnitude of annual average NO2 across monitoring sites, but also the variation in hourly NO2 concentrations that give rise to it (Fig. 1, see Sect. 2.1), and by applying this framework across all sites with available data. This common method of data interpretation allows the relevance of the results from previous studies assessing NO2 variability in one location to be evaluated for other locations. Similarly, the identification of the variability in hourly NO2 concentration contributions to annual average NO2 highlights differences in the likely effectiveness of reducing annual NO2 concentrations through the implementation of mitigation strategies. This is owing to the potential differing impact of mitigation strategies on hourly NO2 concentrations during particular times across the year, and across the day, or the fact that strategies may focus on infrequent peak or frequent moderate hourly NO2 concentrations. Finally, the application of this chemical climatology approach to monitoring data (or modelled data) in other regions would also allow for a consistent comparison of the variability in hourly NO2 contributions to annual average NO2 between regions.
Data for this analysis were obtained from the AirBase European public data repository (https://www.eea.europa.eu/data-and-maps/data/aqereporting-2). Under the EU Air Quality Directive, NO2 must be measured according to the European Standard, EN 14211, 2005 (European Council Directive 2008/50/EC, 2008). This is a chemiluminescence technique involving the reduction of NO2 to NO using heated (300–350 ∘C) molybdenum surfaces followed by the gas-phase reaction between NO and O3. Other measurement methods, including those used in Local Authority air quality monitoring and European Monitoring and Evaluation Programme (EMEP) monitoring, comprise low time resolution NO2 diffusion tube measurement and higher temporal resolution photolytic converter-chemiluminescence NO2 measurements, respectively. Both diffusion tubes and molybdenum converter-chemiluminescence analysers have positive biases in the measurements due to non-specific NO2 chemical measurement (Villena et al., 2012). The photolytic method has fewer artefacts, but at very low NO2 concentration, may have interferences from PAN (Reed et al., 2016). In total, data from 3162 sites monitoring between 2000 and 2014 were assessed against the data capture criteria defined for this analysis (details below). Of the sites which met the data capture criteria, 79 % measured NO2 by chemiluminescence analysers (with molybdenum-converters), 1 % by Differential Optical Absorption Spectroscopy (DOAS), and for the remainder the measurement method was listed as unknown. Data from all available sites in the AirBase repository were utilised in this analysis, as these measurements are used to assess compliance with the EU air quality limit values. The metadata associated with each monitoring site include its location and its classification in terms of general area representativeness (urban, suburban or rural) and station type (traffic, industrial or background) (European Commission, 2013; JRC-AQUILA, 2013). The combination of area and station type produce the classifications used in this paper (e.g. rural background, urban traffic). European monitoring site classification has been evaluated previously (Flemming et al., 2005; Joly and Peuch, 2012; Spangl et al., 2007).
2.1 Chemical climate statistics
The rationale and framework of a “chemical climatology” analysis were outlined in Malley et al. (2014a). The aim is to link a specific impact of atmospheric composition to the conditions that produce it. To this end a chemical climate consists of three elements for which standard sets of statistics are calculated for each site (Fig. 1). First, an impact of atmospheric composition is selected as the focus of the chemical climate. Here the impact is the risk of health effects from long-term exposure to NO2, as quantified through annual average NO2 concentration (henceforth abbreviated to NO2AA), in line with the EU regulatory guidelines. The state of atmospheric composition describes the underlying distributions of hourly NO2 concentrations that contribute to NO2AA. For this analysis, the percent contribution to NO2AA from each month of the year and hour of the day was calculated. In addition, hourly NO2 concentrations for each year at each site were divided into 5 µg m−3 bins (i.e. 0–5, 5–10, 10–15 ... µg m−3) and the percent contribution to NO2AA from each of these bins was calculated to investigate the relative contribution of low, moderate and high NO2 concentrations to NO2AA. These state statistics help to indicate likely causal drivers producing the impact metric, which, for NO2AA, include emissions, meteorology, and atmospheric chemistry (see Sect. 4). To characterise present-day health-relevant NO2 “chemical climates” across Europe, but to average out the influence of any inter-annual variation in annual NO2 concentrations, the statistics shown in Fig. 1 were averaged between 2010 and 2014. Long-term trends in the statistics were evaluated for the period 2000 and 2014, for sites with sufficient data capture during this 15-year period.
At each site NO2AA was calculated if there were > 75 % hourly observations in that year. The 2010–2014 average was calculated if more than three of the five years had valid NO2AA values. These sites were then grouped into 10 µg m−3 bins of average 2010–2014 NO2AA concentration, in order to separately evaluate the conditions associated with very low NO2AA concentrations (i.e. between 0 and 10 µg m−3), through intermediate NO2AA concentrations (i.e. 10–20, 20–30 µg m−3 ...), to the highest observed NO2AA concentrations (> 80 µg m−3).
The data capture for each hour of the day, and each month of the year, was also calculated for each year at each site. For those sites in each of the 2010–2014 NO2AA bins with > 75 % data capture in each month and each hour, the percent contribution of hourly NO2 concentrations in each of the 12 months, in each of the 24 h of the day, and from hourly NO2 concentrations across the year in 5 µg m−3 bins (i.e. from hourly NO2 concentrations between 0–5, 5–10, ... 195–200, > 200 µg m−3) to NO2AA were calculated. These sites were further analysed to identify the major differences in the percentage contribution to NO2AA from each month of the year, hour of the day, and hourly NO2 concentrations in 5 µg m−3 bins across sites with similar 2010–2014 NO2AA values. The relatively low number of sites in the 70–80 and > 80 µg m−3 bins meant that variation in these statistics was examined across the sites individually. However, for the lower NO2AA concentration bins, which contained up to several hundred sites, Ward's hierarchical cluster analysis was used to group sites according to similarity in 77 variables, i.e. the percent contribution to 2010–2014 NO2AA of different months (12 values), hours of the day (24 values), and 5 µg m−3 hourly NO2 concentration bins (41 values, percent contribution from hourly NO2 concentrations 0–5, 5–10, ... 195–200, > 200 µg m−3). In this process, each monitoring site initially constituted its own cluster. Clusters were then merged based on the similarity of the 2010–2014 average values of the percent contribution to NO2AA from January to December, from 00:00 to 23:00 LT, and from each 5 µg m−3 hourly NO2 concentration bin (i.e. the two most “similar” clusters were merged). Euclidean distance was used as the measure of the similarity between these variables between clusters. At each step, the two clusters were merged which resulted in the smallest increase in within-cluster variance, and this was repeated until all sites were contained within a single cluster (Kaufman and Rousseeuw, 1990; Ward, 1963). Other hierarchical and non-hierarchical clustering algorithms and distance measures could have been used in place of the Ward's hierarchical algorithm, and the Euclidean distance, respectively, that were applied in this work. Selection of alternative methods could influence the clustering result (Kaufman and Rousseeuw, 1990), but the Ward's hierarchical cluster algorithm applied here has been shown, in this study, to perform effectively compared with alternative approaches, including with respect to the dispersion of observations, the presence of outliers, and irrelevant variables (Mangiameli et al., 1996).
The clustering of monitoring sites in each 10 µg m−3 2010–2014 NO2AA bin process is represented by a dendrogram, which was then “cut” at an appropriate level to determine the number of clusters, and the grouping of sites. The cut-off point was determined from the visual identification of “elbows” in the plots of the proportion of variance explained against the number of clusters into which sites were divided. The aim in selecting the number of clusters of sites was to maximise the explained variance in the percentage contribution of each month, hour of day, and hourly NO2 concentrations in 5 µg m−3 bins to NO2AA between sites with as few clusters as possible. Therefore, for each 10 µg m−3 2010–2014 NO2AA bin, the point at which increasing the number of clusters resulted in relatively small increases in the proportion of variance explained was identified. The cut-off points selected grouped sites into between five and eight clusters. A key feature of the clustering result is the proportion of variance across sites in the percent contribution to NO2AA from months, hours of the day, and hourly NO2 concentration bins that is explained by dividing sites into a certain number of clusters. The clusters produced in this work explained between 57 and 75 % of the variance between sites in the 10 µg m−3 2010–2014 NO2AA bin ranges, as shown in Fig. S1 in the Supplement. This means that a divergence of between 43 and 25 % remains in these variables between sites with similar 2010–2014 NO2AA which is not explained by dividing sites into the clusters produced in this analysis. Other key aspects of the clustering are the location of sites included in each cluster, and the similarities and differences in the value of each variable (i.e. the percent contribution from each month, hour of day and 5 µg m−3 hourly NO2 concentration bins) between clusters. These aspects are described in Sect. 3.2.
Table 1The number and classification of sites for which 2010–2014 average annual NO2 concentrations were calculated, separated into 10 µg m−3 bins.
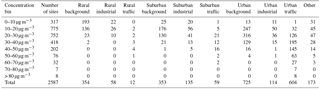
Finally, sites in each of the groups which had long-running NO2 measurements between 2000 and 2014 were identified. Across the 15-year period, a valid statistic (i.e. that met the data capture criteria outlined above) was required in at least 12 of the 15 years for the trend in that statistic to be estimated. The magnitude, direction, and significance of trends in NO2AA, and in month, hour of day, and hourly concentration contributions to NO2AA were calculated using two methods that accounted for autocorrelation in each statistic, but differed in assumptions about the type of underlying distribution of each statistic at each site. A number of parametric and non-parametric trend analysis methods were used to assess the consistency of the results when different methods typically found in the literature for environmental trend analysis were applied. Firstly, the magnitude and direction of the trend was estimated using the non-parametric Theil–Sen statistic, i.e. the median of the trends between all pairs of data points (Theil, 1950a, b, c). The significance of the trend was assessed using block bootstrap resampling to account for autocorrelation in each statistic at each site, using algorithms implemented in the openair package within the R statistical software (Carslaw and Ropkins, 2012; R Core Team, 2016). The block length used was n1∕3 (n is length of time series), the default in the openair package based on the analysis in Kunsch (1989). Secondly, a parametric first-order auto-regressive (AR(1)) model was fitted to each statistic at each site, which also accounts for autocorrelation in the data (Box and Jenkins, 1976). Trend estimations that do not account for autocorrelation in the data were also used. The non-parametric Mann–Kendall statistic was used to estimate the significance of the trend in each statistic at each site (Mann, 1945), in combination with the Theil–Sen statistic for the magnitude and direction of the trend. Finally, trends were also estimated using parametric ordinary least-squares linear regression. These two methods did not account for autocorrelation in the trend estimation, and were therefore used to compare the consistency of results obtained with the non-parametric (Theil–Sen/black bootstrap) and parametric (AR(1)) methods that did account for auto-correlation. All methods used in this work only estimated linear trends, and trends with p ≤ 0.05 were designated as statistically significant. Trends between 2000 and 2014 were assessed by grouping all qualifying sites based on the 2010–2014 NO2AA value at each site. These groupings were used to investigate whether changes in drivers of NO2 variability (e.g. European-wide NOx emission reductions) had different effects at sites where the magnitude of annual NO2 concentrations during the most recent period (2010–2014) differed.
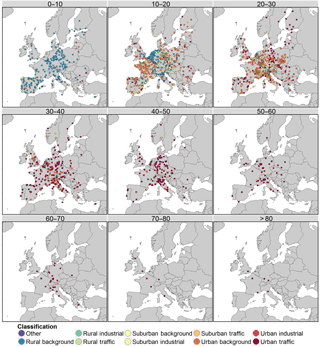
Figure 2Locations of NO2 monitoring sites across Europe. Sites are grouped into 10 µg m−3 concentration bins based on 2010–2014 average annual NO2 concentration, and are coloured according to the EU AirBase site-type classification.
Table 2The number of sites in each 2010–2014 average annual NO2 (NO2AA) concentration bin with sufficient data capture to calculate monthly, hour of day, and hourly concentration contributions to 2010–2014 NO2AA, split by the clusters demarcating distinct variation in monthly, hour of day, and hourly NO2 concentration bin contributions to NO2AA.
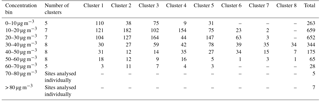
3.1 Impact metric: annual average NO2 concentration
Across Europe, 2587 sites met the data availability criteria to determine the 2010–2014 NO2AA concentration (Table 1), of which 8 % exceeded the 40 µg m−3 EU annual NO2 limit value (Fig. 2). These sites were spread across Europe with 22 out of the 34 countries represented having at least one site where 2010–2014 NO2AA exceeded 40 µg m−3. Most of these exceedances were at urban traffic sites (77 %), consistent with a previous analysis by EEA (2016a). The 2010–2014 NO2AA concentration exceeded 40 µg m−3 at over 50 % of urban traffic sites in Belgium, Germany, Italy, Norway, Serbia, and Switzerland; and at over 40 % of such sites in France, Greece, Portugal and the UK. There were also 20 urban background sites spread across cities in the UK (London, Manchester), Germany (Frankfurt), France (Paris), Spain (Madrid, Barcelona), and Italy (Rome, Monza) where 2010–2014 NO2AA was > 40 µg m−3. However, most urban background sites had 2010–2014 NO2AA concentrations between 10–20 (34 % of all urban background sites), 20–30 (44 %) and 30–40 µg m−3 (18 %). In contrast, most sites with the lowest concentrations were rural background sites; 61 % of sites with 2010–2014 NO2AA concentrations in the 0–10 µg m−3 bin were rural background, and 93 % of all rural background sites had NO2AA concentrations < 20 µg m−3.
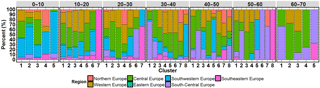
Figure 3The proportion of sites in each 2010–2014 average annual NO2 (NO2AA) 10 µg m−3 concentration bin in different European regions (shown in Fig. S2), separated by clusters demarcating distinct variation in monthly, hour of day, and hourly NO2 concentration bin contributions to NO2AA.
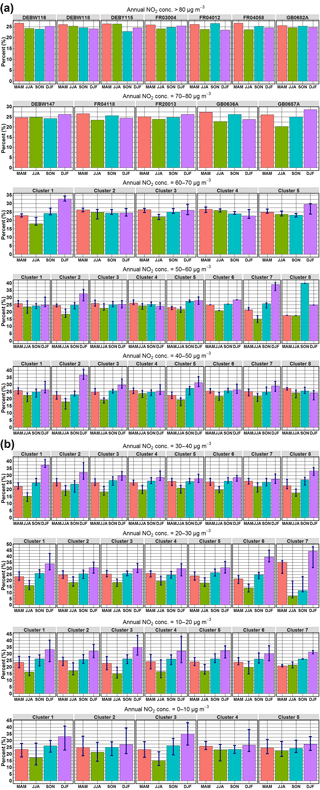
Figure 4The contribution to 2010–2014 average annual NO2 concentrations (NO2AA) from spring (March, April, and May), summer (June, July, and August), autumn (September, October, and November), and winter (December, January, and February) months for sites with 2010–2014 NO2AA concentrations for sites between (a) 40 and > 80 µg m−3 and between (b) 0 and 40 µg m−3, split into 10 µg m−3 bins based on the magnitude of the 2010–2014 NO2AA concentration. For the > 80 and 70–80 µg m−3 bins, these contributions are shown for sites individually (identified by Airbase ID), while for lower concentration bins sites are grouped into clusters with distinct variation in monthly, hour of day, and hourly concentration contribution to NO2AA. For these bins, the height of the bar shows the median contribution to 2010–2014 NO2AA from that season across all sites, with the 5th and 95th percentile values across the sites shown as uncertainty bars.
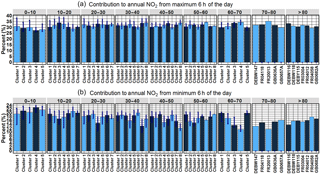
Figure 5The contribution to 2010–2014 average annual NO2 concentrations (NO2AA) from (a) the 6 h with the largest contribution to 2010–2014 NO2AA, and (b) the 6 h with the smallest contribution to 2010–2014 NO2AA, for sites separated by 2010–2014 NO2AA concentrations, and clusters demarcating distinct variation in monthly, hour of day, and hourly NO2 concentration bin contributions to NO2AA. These statistics are a proxy for variation in contribution to NO2AA across the diurnal cycle.
3.2 State: monthly, hour of day, and hourly concentration bin contribution to annual average NO2
Of the 2587 sites that had sufficient data capture to calculate 2010–2014 NO2AA concentrations, 2198 (85 %) had sufficient data capture (> 75 %) in each month of the year and each hour of the day to assess hourly NO2 contributions to NO2AA (Table 2). This included seven of the eight sites with 2010–2014 NO2AA concentrations > 80 µg m−3, and five of the seven sites with NO2AA between 70–80 µg m−3. As described in Sect. 2, the large number of sites with 2010–2014 NO2AA in each of the lower concentration bins were clustered according to similarity in monthly, hour of day, and hourly NO2 concentration bin contributions to NO2AA (summarised in Table 2). The proportion of sites located in different regions of Europe assigned to each cluster is shown in Fig. 3 (regions are defined in Fig. S2), and the locations of the sites in each cluster for each NO2AA bin are shown in Figs. S3–S9. For the majority of clusters across the 2010–2014 NO2AA bins, the sites were geographically widespread across Europe. This indicates that the distinct variations in monthly, hour of day, and hourly NO2 concentration bin contributions to NO2AA identified by the cluster analysis are not confined to specific European regions, e.g. for the 60–70 µg m−3 2010–2014 NO2AA bin, sites in Central Europe made up a substantial proportion of sites in four of the five clusters (Fig. 3). However, there were also clusters that did not contain sites in particular Europe regions, and clusters that were dominated by sites from one region. Most notably there were clusters in each NO2AA bin between 20–30 and 60–70 µg m−3 that predominantly contained sites from northern Italy and neighbouring areas. The distinct variations in the contribution of months, hour of day and hourly NO2 concentration bins that lead to cluster separation have been summarised as (i) the differences in seasonal contribution to NO2AA, as shown in Fig. 4a and b; (ii) the contribution from the 6 hours with the largest contribution to NO2AA, and the 6 h with the lowest contribution to NO2AA, as a proxy for the amplitude of diurnal variation in hour of day contributions to NO2AA (Fig. 5); and (iii) the contribution from the five, ten, and fifteen 5 µg m−3 hourly NO2 concentration bins with the largest contribution to NO2AA, as a proxy for the breadth of hourly NO2 concentrations that make a substantial contribution to NO2AA (Fig. 6).
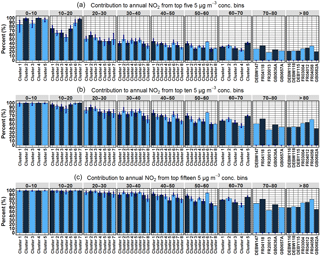
Figure 6The contribution to 2010–2014 average annual NO2 concentrations (NO2AA) from the (a) five, (b) ten, and (c) fifteen 5 µg m−3 hourly NO2 concentration bins with the largest contribution to 2010–2014 NO2AA, for sites with 2010–2014 NO2AA in 10 µg m−3 bins, and separated by clusters demarcating distinct variation in monthly, hour of day, and hourly NO2 concentration bin contributions to NO2AA. These values are a proxy to compare the range of hourly NO2 concentrations contributing to NO2AA, and the near 100 % contribution for the lower 2010–2014 NO2AA bins results from the fact that for NO2AA of 0–10 µg m−3, there are far fewer high hourly NO2concentration values.
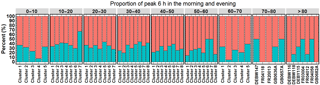
Figure 7Proportion of the 6 h of the day that make the largest contribution to 2010–2014 average annual NO2 concentrations (NO2AA) that occurred in the morning (before 12:00, blue), and in the afternoon/evening (after 12:00, red), for sites with 2010–2014 NO2AA in 10 µg m−3 bins, and separated by clusters demarcating distinct variation in monthly, hour of day, and hourly NO2 concentration bin contributions to NO2AA.
The majority of sites where 2010–2014 NO2AA exceeded 40 µg m−3 were between 40 and 70 µg m−3. However, analysis of the conditions producing 2010–2014 NO2AA at the sites with highest 2010–2014 NO2AA (i.e. > 70 µg m−3) illustrates patterns that also occurred across the majority of sites that exceeded the EU limit value. All sites with the highest 2010–2014 NO2AA concentration (> 80 µg m−3) lacked seasonal variation in the contribution of hourly NO2 concentrations to NO2AA (Fig. 4a) and had largest contributions to NO2AA from daytime hours, with morning and evening peaks, reflecting their urban traffic classification. Whilst the 30–35 % contribution to NO2AA from peak hours (Fig. 5), defined as the 6 h with largest contribution to NO2AA, was similar across these sites, the timing of the peak periods differed, e.g. due to differences in the magnitude of the morning and evening peaks in NO2 concentration. For example, the top 6 h occurred exclusively during the afternoon/evening at two of the highest NO2AA sites, five of the six peak hours occurred in the evening at two sites, four out of six peak hours occurred in the evening at one site, and two sites had an equal number of top 6 h during the morning and evening (Fig. 7).
In addition, the contribution from different hourly NO2 concentration bins to NO2AA varied across these sites with highest NO2AA. At the three French sites, a narrower range of 5 µg m−3 concentration bins made substantial contributions to NO2AA compared to the three German sites and one UK site. This is illustrated by the larger contribution of the top five contributing 5 µg m−3 hourly NO2 bins (27–34 % of NO2AA) at the three French sites, compared to the German and UK sites (21–22 %) (Fig. 6). Consequently, while NO2AA was similar across these sites, the frequency of short-term peak NO2 concentrations was not. Hourly NO2 concentrations above the 200 µg m−3 hourly NO2 EU limit value contributed 6 % to NO2AA at GB0682A, and 2–4 % at the three German sites, compared with only 0.3–1 % at the French sites. Controlling hourly EU limit value exceedances would therefore have a greater impact on reducing NO2AA at the UK and German sites compared with the French sites. Conversely, reduction in the relatively moderate hourly NO2 concentrations (60–120 µg m−3) would yield greater reductions in NO2AA at the French sites (where contribution from 60–120 µg m−3 to NO2AA varied between 60 and 68 %) compared to the German and UK sites (45–50 %). The patterns of variation in contribution from months, hour of day, and hourly NO2 concentrations at the sites with 2010–2014 NO2AA between 70 and 80 µg m−3 were broadly similar to those identified for the > 80 µg m−3 sites (Figs. 4–6).
As shown in Sect. 3.1 the majority of sites where 2010–2014 NO2AA exceeded 40 µg m−3 were between 40 and 70 µg m−3. For sites with 2010–2014 NO2AA in the range 60–70 µg m−3, most were urban traffic sites (Fig. 2), and 61 % were grouped into two of the five clusters identified (Cluster 2 and Cluster 3). These sites were geographically widespread (Figs. 3, S3), and had similar patterns of hourly NO2 contribution to NO2AA as at sites with higher 2010–2014 NO2AA. There was little variation in seasonal contribution to NO2AA (Fig. 4a), a 30–35 % contribution in the top 6 h of the day to NO2AA, and similar variation in the contribution of hourly NO2 concentration bins to NO2AA. Cluster 2 sites had lower diurnal variation in the contribution to NO2AA, as shown by a relatively lower contribution from the peak 6 h of the day to NO2AA (31 % compared with 34 % for Cluster 3), and a higher contribution from the minimum 6 hours of the day compared with Cluster 3 (17 % vs. 13 %). In addition, sites in Cluster 2 were determined by a relatively narrower set of hourly NO2 concentrations, with the top five contributing 5 µg m−3 hourly NO2 bins responsible for 34 % of NO2AA, compared with only 28 % of NO2AA for Cluster 3 sites (Fig. 6).
Other clusters contained sites with 2010–2014 NO2AA between 60 and 70 µg m−3 where the conditions producing NO2AA were distinct to those sites with higher NO2AA (and sites in Clusters 2 & 3). For example, the three sites in Cluster 1 were in northern Italy and western Austria, and had substantial seasonal variation in contribution to NO2AA (Fig. 4a), with 33 % of NO2AA derived during winter, and only 18 % during summer. There was also less diurnal variation in contribution to NO2AA than for sites in Cluster 2 and 3, with the peak 6 h contributing 30 %, and the minimum 6 h 20 % (Fig. 5). In contrast, the distinction between the three sites in Cluster 4, and others was the larger diurnal variation in contribution to NO2AA, with the peak 6 h contributing 34 %, and the minimum 6 h only 11 %. The Cluster 4 sites, in southern Germany and northern Italy, also had the broadest range of concentrations contributing to NO2AA, with only 24 % of NO2AA accounted for by the top five 5 µg m−3 hourly NO2 bins.
Sites with NO2AA in the range 50–60 µg m−3 were grouped into 8 clusters, with the majority (85 %) grouped into four clusters (Table 2). As for the 60–70 µg m−3 sites, one cluster (Cluster 2) included sites predominantly in northern Italy (as well as Switzerland, southern France, and Hungary), and which had substantial seasonal variation in contribution to NO2AA (Fig. 4a). In contrast the sites in the other 3 major clusters (Clusters 1, 3, and 4, containing 66 % of sites) were more similar to those sites with higher NO2AA (> 70 µg m−3), with little seasonal variation in contribution to NO2AA. The distinctions between sites in these three clusters were the extent of variation in diurnal contribution to NO2AA (Cluster 1 sites had the smallest difference in contribution from the peak and minimum 6 h, Cluster 3 had the largest; Fig. 5), and range of hourly NO2 concentrations that contributed to NO2AA (Cluster 1 sites had the narrowest range, Cluster 3 sites the widest). Sites with 2010–2014 NO2AA between 50 and 60 µg m−3 in each of these major clusters were quite geographically widespread, and located in cities throughout Europe.
For sites with 2010–2014 NO2AA between 40 and 50 µg m−3, there was also a cluster (Cluster 2) with sites in northern Italy and Austria and which had the largest seasonal variation in contribution to NO2AA (Fig. 4a). Sites in other clusters (Clusters 3 and 5) also had relatively large winter contribution to NO2AA compared with most sites with higher NO2AA, and these sites were located across central and southern Europe, rather than confined to northern Italy (Figs. 3, S5). However, many sites with NO2AA in this range, spread across a large number of countries, also showed little seasonal variation in contribution to NO2AA. These sites grouped in Clusters 1, 4, and 6, and were distinguished by relatively low diurnal variation and narrower range of hourly NO2 concentrations determining NO2AA for sites in Clusters 1 and 6 compared to sites in Cluster 4.
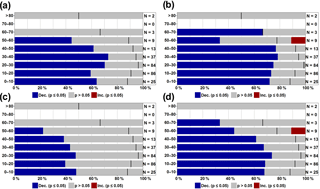
Figure 8Proportion of monitoring sites with 2010–2014 average annual NO2 concentrations (NO2AA) in 10 µg m−3 bins that had significant decreasing (blue), significant increasing (red) (p ≤ 0.05), and non-significant trends (grey) in NO2AA between 2000 and 2014, calculated using (a) non-parametric Theil–Sen statistic and Mann–Kendall test, (b) parametric ordinary least-squares linear regression, (c) non-parametric Theil–Sen statistic and block bootstrap resampling, and (d) parametric first-order autoregressive (AR(1)) model. The black line represents the division between decreasing and increasing trends within the non-significant bar.
Although at the majority of sites the 2010–2014 NO2AA concentration did not exceed the 40 µg m−3 EU limit value, negative health outcomes may be associated with lower concentrations (REVIHAAP, 2013). For NO2AA < 40 µg m−3, sites across all clusters tended to have larger contributions from winter to NO2AA and lower contributions from summer. As expected, as NO2AA decreased there was also a narrower range of hourly NO2 concentrations that contributed to NO2AA, and therefore the top 5, 10, and 15 largest contributing concentration bins contributed a larger proportion of NO2AA than at sites with higher NO2AA (Fig. 6). There were also clusters of sites in specific locations with distinct hourly NO2 concentration contributions to NO2AA. For example, in the 30–40 and 20–30 µg m−3 2010–2014 NO2AA bins, there were clusters containing sites in northern Italy and neighbouring areas (Cluster 1 and Cluster 6, respectively) that had the largest seasonal difference in contribution to NO2AA across all clusters in these 2010–2014 NO2AA bins. Similar to the sites with higher NO2AA concentrations, the clustering of sites with 2010–2014 NO2AA < 40 µg m−3 grouped sites from across wide geographic areas based on (i) a relatively narrow distribution of hourly NO2 concentrations contributing to NO2AA (e.g. 30–40 µg m−3, Cluster 2; 20–30 µg m−3, Cluster 4, Fig. 6), or (ii) a relatively large diurnal variation in contribution to NO2AA (e.g. 30–40 µg m−3, Cluster 4 and Cluster 7; 20–30 µg m−3, Cluster 4, Fig. 5). In addition, the contribution from morning and evening hours to those hours of the day with peak contribution also varied, as was the case for the sites with higher NO2AA (Fig. 7).
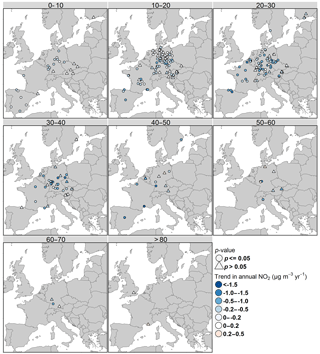
Figure 9Magnitude and significance of trend in annual average NO2 concentrations between 2000 and 2014, with sites separated into panels based on 2010–2014 average annual NO2 concentrations (NO2AA). The colour in each point denotes the magnitude and direction of the Theil–Sen trend at a site, and the outer colour denotes whether the trend was statistically significant (p ≤ 0.05, circle), or not statistically significant (p > 0.05, triangle). Trend estimates were calculated using a first-order autoregressive (AR(1)) model.
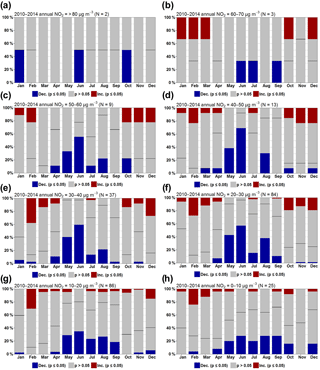
Figure 10Proportion of sites with significant decreasing (blue), increasing (red) (p ≤ 0.05), and non-significant (grey) trends in the monthly percentage contribution to annual average NO2 between 2000 and 2014, for sites with 2010–2014 average annual NO2 concentrations (NO2AA) of (a) > 80 µg m−3, (b) 60–70 µg m−3, (c) 50-60 µg m−3, (d) 40–50 µg m−3, (e) 30–40 µg m−3, (f) 20–30 µg m−3, (g) 10–20 µg m−3, and (h) 0–10 µg m−3. Trend estimates were calculated using a first-order autoregressive (AR(1)) model. The black line represents the division between decreasing and increasing trends within the non-significant bar.
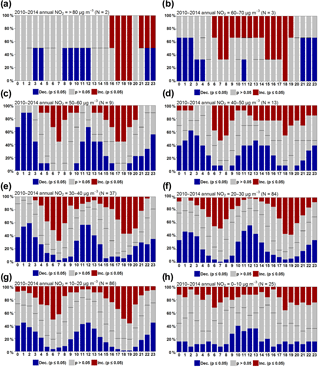
Figure 11Proportion of sites with significant decreasing (blue), increasing (red) (p ≤ 0.05), and non-significant (grey) trends in the percentage contribution of each hour of day to annual average NO2 between 2000 and 2014, for sites with 2010–2014 average annual NO2 concentrations (NO2AA) of (a) > 80 µg m−3, (b) 60–70 µg m−3, (c) 50–60 µg m−3, (d) 40–50 µg m−3, (e) 30–40 µg m−3, (f) 20–30 µg m−3, (g) 10–20 µg m−3, and (h) 0–10 µg m−3. Trend estimates were calculated using a first-order autoregressive (AR(1)) model. The black line represents the division between decreasing and increasing trends within the non-significant bar.
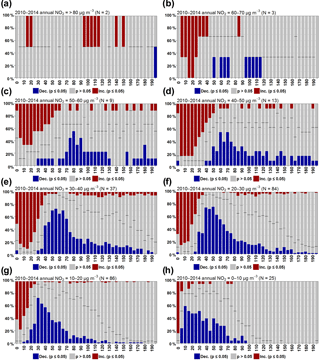
Figure 12Proportion of sites with significant decreasing (blue), increasing (red) (p < 0.05), and non-significant (grey) trends in the percentage contribution from hourly NO2 concentrations in 5 µg m−3 bins to annual average NO2 between 2000 and 2014, for sites with 2010–2014 average annual NO2 concentrations (NO2AA) of (a) > 80 µg m−3, (b) 60–70 µg m−3, (c) 50-60 µg m−3, (d) 40–50 µg m−3, (e) 30–40 µg m−3, (f) 20–30 µg m−3, (g) 10–20 µg m−3, and (h) 0–10 µg m−3. Trend estimates were calculated using a first-order autoregressive (AR(1)) model. The black line represents the division between decreasing and increasing trends within the non-significant bar.
3.3 Long-term trends
Trends between 2000 and 2014 were calculated at 259 sites. The estimated trends using parametric and non-parametric approaches, and methods which did and did not account for autocorrelation produced generally consistent results for the changes in NO2AA and monthly, hour of day, and hourly NO2 concentration bin contributions to NO2AA. Figure 8 shows the proportion of sites with significant decreasing, increasing and non-significant trends in NO2AA between 2000 and 2014. The proportion of sites in each 2010–2014 NO2AA bin with significant decreasing trends was similar for the Theil–Sen/Mann–Kendall (Fig. 8a), linear regression (Fig. 8b) and first-order autoregressive (AR(1)) (Fig. 8d) methods, and 20–30 % lower for the Theil–Sen/block bootstrap method (Fig. 8c). However, the magnitude and direction of the trends in NO2AA across all sites were very similar for the Theil–Sen/block bootstrap or AR(1) methods (Fig. S10). The spatial distribution of 2000–2014 changes in NO2AA is shown in Fig. 9, and the proportion of sites in each 2010–2014 NO2AA bins with significant decreasing, increasing (p ≤ 0.05), and non-significant trends in monthly, hour of day, and hourly NO2 concentration bin contribution to NO2AA are shown in Figs. 10, 11 and 12, respectively. These figures show the parametric AR(1)-estimated trends because, in contrast to linear regression, autocorrelation was adjusted for using this method. The corresponding summaries of the non-parametric Theil–Sen/block bootstrap-derived trend estimates are provided in the Supplement for comparison, and show broadly similar patterns (Figs. S11–S14). The statistics referred to below are from the AR(1) trend estimation.
The estimated trend in NO2AA was negative at the majority of sites. For sites where 2010–2014 NO2AA exceeded 40 µg m−3, the trend was not statistically significant at a larger proportion of sites (54 %, i.e. 14 out of 27 sites) than those with lower 2010–2014 NO2AA (Fig. 8d). Sites with 2010–2014 NO2AA concentrations in the ranges 30–40, 20–30, 10–20, and 0–10 µg m−3 had significant decreasing trends in NO2AA at 68, 74, 69, and 68 % of sites, respectively (Fig. 8). Although most sites had a non-significant change in monthly contribution to NO2AA between 2000 and 2014, there was a larger proportion of sites with a significant decreasing trend in the contribution to NO2AA from summer months, in particular from June, and significant increasing contribution from winter months (Fig. 10). The predominant pattern of change in hour of day contribution to NO2AA was an increasing contribution from “peak” morning and evening hours, and a decreasing contribution to NO2AA from night-time hours, and hours in the middle of the day (Fig. 11). For sites in the lowest 2010–2014 NO2AA category (0–10 µg m−3), a smaller proportion of sites had significant increasing or decreasing trends in the contribution across the day compared to sites with highest 2010–2014 NO2AA. Finally, across all 2010–2014 NO2AA bins, the predominant pattern of change in the contribution of hourly NO2 concentrations to NO2AA between 2000 and 2014 was an increasing contribution from relatively low hourly NO2 concentrations, and a decreasing contribution from relatively high hourly NO2 concentrations (Fig. 12).
The calculation of a standard set of statistics for thousands of monitoring sites showed that sites with similar NO2AA differed in the contribution from hourly NO2 concentrations during different months, hours of the day, and across the hourly NO2 concentration distribution. Clustering of sites based on these distinct contributions to NO2AA showed that sites with similar NO2AA located relatively close to one another were often grouped into different clusters, and that sites relatively far from one another were often grouped within the same clusters (Fig. 3). The observations that multiple distinct patterns contributed to NO2AA in a particular European region builds on analysis by Cyrys et al. (2012) showing that variation in NO2AA within a particular city was greater than variation in NO2AA across the 36 cities analysed. The present study shows that there can also be variation in the contributions from months, hours of the day, and hourly NO2 concentration bins to NO2AA within relatively small geographic areas across Europe
4.1 Drivers: links to meteorology, emissions, and chemistry
The specific location of a monitoring site determines the relative contributions of meteorological conditions, atmospheric chemistry and NOx emission sources to the NO2AA concentration. In this work, sites with 2010–2014 NO2AA above 40 µg m−3 were almost all urban traffic sites (JRC-AQUILA, 2013). At these sites, the lack of a seasonal pattern in contribution to NO2AA indicates that the major driver for NO2AA is the NOx emission sources in close proximity to the site. In addition to primary NO2 emissions, the presence of O3 at these urban traffic sites will also lead to secondary formation of NO2 (from primary NO emissions). This reaction can proceed in a few seconds at polluted locations (AQEG, 2004), within the timescale of dispersion from source to roadside monitor, and suggests that long-range transport does not play the major role in driving exceedance of the NO2AA EU limit value. These conditions occur in cities across Europe. Schäfer et al. (2006) also showed in Hanover, Germany, that pollutant concentrations closest to major roads in street canyons were substantially less correlated with boundary layer mixing height compared with urban background stations. Hence NO2AA at these sites may also be less influenced than sites with lower NO2AA by seasonal differences in boundary layer height, which are generally lower during the day in winter (Seidel et al., 2012). Reductions in hourly NO2 concentrations across the whole year, e.g. through local NOx emission reductions, would therefore yield similar benefits in reducing NO2AA for the majority of sites exceeding the EU NO2 annual limit value, rather than just in winter months, owing to the relatively equal contribution from hourly NO2 concentrations during all seasons (Fig. 4a). However, the extent of the reduction from reducing emissions during peak hours of the day, and from reducing the short-term peak hourly NO2 concentrations, varies between sites, which may reflect variability between sites in meteorological conditions or in traffic flows (i.e. strength of the emission source in close proximity to the monitoring sites).
Some sites, generally located in northern Italy and neighbouring areas, with 2010–2014 NO2AA between 40 and 70 µg m−3 had substantially larger contributions from winter months to NO2AA. This indicates that in this region the photochemical conversion of NO2 to NO and O3 in summer may be a more important factor in determining NO2 concentrations (i.e. lowering NO2 concentration during summer) at these sites than at other sites across Europe with similar NO2AA concentrations. Northern Italy is a heavily industrialised and relatively polluted region of Europe (Carugno et al., 2016; Cyrys et al., 2012; Hazenkamp-Von Arx et al., 2004). Large NOx and volatile organic compound (VOC) emissions in this region, combined with relatively high levels of solar radiation in summer, have previously been factors attributed to high levels of summertime photochemical ozone production (Masiol et al., 2017). Hence reducing hourly NO2 concentrations during the winter would be more effective in reducing NO2AA, but reducing NOx emissions during the summer could reduce photochemical O3 production in northern Italy and neighbouring areas. In addition to changes in atmospheric photochemistry between seasons, higher wintertime NO2 concentrations have also been attributed to higher emissions from residential heating, and seasonal changes in meteorological conditions (Henschel et al., 2016). For example, Palarz et al. (2017) calculated the frequency of temperature inversions at 00:00, 06:00, 12:00, and 18:00 UTC across Europe during different seasons between 1981 and 2015. In winter, southern Europe, including northern Italy, had the highest frequency of temperature inversions at 00:00, 06:00, and 18:00 UTC across mainland Europe. However, in summer, temperature inversions were less common in northern Italy at 06:00 UTC compared to south-western Europe (Iberian Peninsula), and at 18:00 UTC compared to south-eastern Europe, i.e. in summer meteorological conditions were such that temperature inversions tended to dissipate earlier in northern Italy compared to the Iberian Peninsula, and form later than in south-eastern Europe. This indicates that, compared to the rest of Europe, in northern Italy there may be a larger difference between winter and summer in the frequency of temperature inversions and the trapping of pollution close to the surface that may contribute to the large difference in seasonal contribution to NO2AA at sites in this region.
There was also a greater contribution from winter compared to summer months to NO2AA for the majority of sites across Europe with NO2AA below the EU limit. This likely reflects the greater distance of these sites from direct NOx emission sources, given that most of them were not urban traffic sites. The longer timescales of dispersion become more important in controlling NO2 concentrations at these sites, and during summer NO2 can be photolysed to NO and O3 during transport from source to receptor. Pollutant concentrations at urban sites further from emissions sources were also shown to be more dependent on boundary layer height compared to traffic sites (Schäfer et al., 2006), which during the day are higher in summer compared to winter (Seidel et al., 2012), increasing the volume into which NOx emissions can be dispersed.
The conditions producing NO2AA have changed since 2000, even though at some sites this has not resulted in a statistically significant change in NO2AA. Between 2000 and 2014, NOx emissions from all sources were estimated to have decreased by 39 %, and by 46 % for road transport specifically (EEA, 2016b). However, Carslaw et al. (2011, 2016) showed that road transport NOx emission decreases may not have been as large as expected from the implementation of vehicle emission standards. Carslaw et al. (2016) furthermore showed, at roadside sites in London, that reductions in NO2 concentrations had been smaller than decreases in NOx concentrations between 1995 and 2015, and the NO2 ∕ NOx emission ratio increased from less than 5 % in 1997, to 25 % in 2010, and 15 % in 2014. The lower ratio in 2014 was attributed to a reduction in NO2 ∕ NOx ratios in NOx emissions from heavy duty vehicles as a result of Euro IV and Euro V vehicle emissions standards. A similar increase in the NO2 ∕ NOx emission ratio was also shown across the EU by Grice et al. (2009). The lower than estimated reduction in NOx emissions from road transport, and the increase in the fraction of NOx emitted as NO2, may be factors contributing to non-significant trends in NO2AA estimated at some sites, especially at the urban traffic sites with highest 2010–2014 NO2AA.
The major changes in the hourly NO2 contributions to NO2AA between 2000 and 2014 were a decreasing contribution from summer months, and an increasing contribution from winter months, an increasing contribution from hours during peak road traffic hours, and decreasing contributions from the highest hourly NO2 concentrations. Henschel et al. (2016) analysed changes in seasonal NO2 concentrations in nine European cities and reported a decrease in summer NO2 concentrations, and an increase in winter concentrations, which is consistent with the seasonal contribution trends calculated here. The present analysis indicates that the factors contributing to enhanced wintertime NO2 concentrations have become more important in determining NO2AA between 2000 and 2014 at the majority of sites. This includes larger NOx emissions from building heating during winter (Henschel et al., 2016), and meteorological conditions, with shallower daytime boundary layers (Seidel et al., 2012) and more frequent, deeper and stronger daytime temperature inversions in winter compared with summer (Palarz et al., 2017). Palarz et al. (2017) estimated that between 1981 and 2015, the frequency of night-time temperature inversions across most of mainland Europe had not changed significantly in winter or summer, and during summer, there was little change in the depth and strength of these inversions. In contrast, during winter, significant decreasing trends in the depth and strength of temperature inversions were calculated across most of mainland Europe. Reductions in the depth into which emissions can disperse during wintertime temperature inversions may contribute to the increasing contribution of hourly NO2 concentrations during winter to annual NO2 concentrations. However, Palarz et al. (2017) did not estimate trends in the frequency, depth and strength temperature inversions during the daytime, when the majority of NOx emissions occur, and did not specifically assess changes between 2000 and 2014 for which trends were calculated in this work.
The increasing contribution to NO2AA from rush-hour periods between 2000 and 2014 across sites with a wide range of 2010–2014 NO2AA underlines the importance of local road transport NOx emission sources in determining NO2AA at monitoring sites across Europe. The increase in contribution to NO2AA from rush-hour periods may reflect smaller decreases in NO2 concentrations during these hours compared to other hours, lower than expected decreases in total road transport NOx emissions during the period 2000–2014, as well as increases in the fraction of road transport NOx emissions emitted as NO2 (Carslaw et al., 2016).
Finally, the 2000–2014 trends indicate that conditions producing the highest hourly NO2 concentrations have decreased in frequency. This may reflect decreases in NOx emissions overall, but may also reflect changes in traffic patterns in particular cities (Baptista et al., 2014; CEBR, 2014; INRIX, 2016; Thomas, 2016), or meteorological conditions that concentrate NO2 close to the monitoring sites. For example, Wagner and Schäfer (2015) showed that in an urban street canyon there was a substantially stronger relationship between decreasing peak NO2 concentrations and increasing mixing layer height than for mean NO2 concentrations, for which there was no significant relationship. Therefore any increase in mixing layer height may be expected to disproportionately reduce highest hourly NO2 concentrations as opposed to more moderate levels. Zhang et al. (2013) concluded that daytime (12:00 UTC) boundary layer height had increased between 1973 and 2010 based on daily radiosonde observations at 25 stations across Europe (average 73 m decade−1), with no indication of seasonal differences.
Some studies have used satellite data to estimate trends in NO2AA across Europe (Blond et al., 2007; Geddes et al., 2016; Konovalov et al., 2010; Schneider et al., 2015); Geddes et al. (2016) estimate an average 1996–2012 trend in annual NO2 of −2.2 % yr−1. The current analysis decomposes the seasonal, hour of day, and hourly NO2 concentration changes that have produced this average trend, but also highlights variation in the magnitude, and statistical significance of NO2AA trends across Europe. Many sites with 2010–2014 NO2AA exceeding the annual EU limit value had a non-significant trend in NO2AA, which may not be reflected in the satellite derived trend estimates due to the spatial resolution of the satellite NO2 estimates (e.g. 0.1 × 0.1∘ in Geddes et al., 2016).
4.2 Implications for policies aimed at reducing annual NO2 concentrations across Europe
Previous studies have shown that specific mitigation actions aimed at reducing NO2 concentrations can have varying effects on achieving this goal. For example, Jeanjean et al. (2017) modelled the effect of seven mitigation actions on mean NO2 concentrations for a street in London, including additional trees lining the street, physical barriers between the road and pedestrian areas, and paint applied to buildings. The individual implementation of these measures changed mean NO2 concentrations beside roads between a 0.1 % increase and a 7.4 % decrease. Additionally, the effect of some mitigation measures on reducing NO2 concentrations during a particular season, hour of day, or on peak vs. moderate concentrations can vary. For example, NOx emission reduction from reducing indoor building temperatures disproportionately occur in winter when heating is required (Chiesa et al., 2014).
The analysis presented here does not specifically identify the likely effect of individual mitigation actions aimed at reducing annual NO2 concentrations on NO2AA at the different groups of sites identified. However, a greater understanding of the variability in hourly NO2 concentration driving NO2AA at different sites, for which a methodology and practical application is the focus of this paper, increases the ability to assess how changes in hourly NO2 variation at a site would affect NO2AA, e.g. due to a particular mitigation action. For example, total traffic flows in cities in the Netherlands (Almelo), and in Greece (Athens) are lower in July and August compared with winter months, and the distribution of peak traffic flow also differ, with these differences attributed to fewer commutes during the holiday period (Mavroidis and Ilia, 2012; Stathopoulos and Karlaftis, 2001; Thomas et al., 2008; Weijermars, 2007). Measures to reduce congestion in these cities may therefore disproportionately reduce traffic flows during the winter, when congestion is worse.
This work, focusing on linking hourly NO2 variation to annual NO2 concentrations, shows that there are sites across Europe with similar NO2AA, but with distinct variation in seasonal contributions. With this knowledge, the effect of such a policy which disproportionately reduces winter NOx emissions may therefore be more effective at those sites within an NO2AA bin that have larger winter contributions to NO2AA, and at those sites with relatively lower NO2AA, as winter contributions to NO2AA tended to increase as NO2AA decreased. More generally, measures implemented to reduce NO2AA in one location may be less effective in others, and the development of strategies to reduce NO2AA in a particular area should consider the variation in monthly, hour of day, and hourly NO2 concentrations contributions to NO2AA within that area. Similarly, consideration should also be given to how different mitigation actions will affect hourly NO2 concentrations across the year, hour of day, and peak vs. moderate hourly NO2 concentrations, as this will determine the extent to which that measure will be effective at different locations. In summary, if how NO2AA arises at sites across a region is known, then the ability of a monitoring network is enhanced to inform on how changes in hourly NO2 concentrations, resulting from the implementation of a particular mitigation action, will affect an impact or regulatory metric such as annual NO2 concentrations.
4.3 Uncertainties and limitations
A key consideration is whether the sites used in this analysis are representative of the range of contributions from hourly NO2 concentrations producing NO2AA across Europe. As outlined above, even within relatively small geographic areas NO2AA, and contributions from months, hours and hourly NO2 concentration bins to NO2AA, can vary. Hence even though the NO2 monitoring network across EU member states is among the densest monitoring networks in the world, there may be locations where the combination of proximity to emission source, and factors affecting dispersion of NOx emissions mean that the contribution of months, hours of the day, and hourly NO2 concentrations is distinct from the monitored locations within that city. Multiple studies have previously assessed monitoring site representativeness across Europe, using a wide variety of methods, including classifying sites based on population or other non-pollutant proxy variables (Joly and Peuch, 2012; Spangl et al., 2007), and classifying sites based on the similarity of air pollutant concentrations (Austin et al., 2013; Malley et al., 2014b; Tarasova et al., 2007). This analysis shows that, for NO2, sites in close geographic proximity to one another can have different hourly NO2 contributions to annual average NO2, while there can be substantial similarity in those conditions at sites distant from one another. This is in contrast to assessments of site representativeness for other pollutants, such as ozone, where distinct geographic groupings were identified from the analysis of ozone datasets (Malley et al., 2014b; Tarasova et al., 2007). This work therefore emphasises that while there have been multiple methodologies outlined for classifying monitoring sites, one consideration is that a classification based on pollutant measurements is likely to be pollutant-specific, and therefore may differ depending on the pollutant selected to undertake the classification.
The trend analysis undertaken in this work estimated the linear trend in NO2AA, and monthly, hour of day, and hourly NO2 concentration bins contributions. Broadly consistent results were obtained using parametric and non-parametric methods, and with and without adjustment for autocorrelation. The proportion of sites with different trend assignments (i.e. significant increasing, decreasing or non-significant) across the four trend estimates calculated for each statistic differed by 20–30 % at most. This indicates that assumptions about the underlying distribution of the data and the level of autocorrelation did not introduce bias into the trend estimates that might lead to different conclusions on the general patterns of change. However, changes in the drivers of NO2 concentrations have not occurred linearly (Carslaw and Carslaw, 2007), and the trend analysis does not account for these step changes and non-linearities. NO2 concentrations have been shown to be affected by particular events; e.g. the 2008–2009 economic recession (Castellanos and Boersma, 2012; Cuevas et al., 2014), increases in urban buses and diesel vehicles (Carslaw and Carslaw, 2007), or changes in the NO2 ∕ NOx emission ratio (Carslaw et al., 2016). Future work to investigate non-linear changes in the statistics calculated in this work could provide additional insight into how hourly NO2 concentrations determining NO2AA change in response to the implementation of specific policies or mitigation strategies. Additionally, the trend analysis was conducted at sites with sufficient data capture between 2000 and 2014. The 259 sites which met these criteria were not evenly distributed across Europe, but were disproportionately grouped in central Europe, specifically in Germany, where 53 % of the sites were located, followed by Austria (15 %) and France (14 %). Hence the trend results presented here are determined predominantly by the specific changes that have occurred at sites in central Europe, rather than reflecting changes across the whole of Europe. A geographically broader comparison of the consistency of changes in NO2AA, and the conditions producing it across Europe, would be enhanced by the calculation of trends in the statistics derived here at sites in southern, eastern and northern Europe as the length of time series increase at sites in these regions, and by assessment of spatial autocorrelation across sites included in the trend analysis. Finally, assessment of trends between 2000 and 2014 does not indicate how trends at each site are likely to progress into the future, which will be determined by future changes in NOx emissions, and meteorology.
The aim of this analysis was to demonstrate that calculating a standard set of “chemical climate” statistics at a large number of monitoring sites could increase the information gained from the monitoring network. The calculation of a standard set of statistics has shown that, in addition to the variety of NO2AA across European sites shown previously (Cyrys et al., 2012), sites with similar NO2AA across Europe differ in how NO2AA is derived. Hence this study provides information as to how reducing NO2 during specific months of the year, hours of the day, or focusing on peak vs. moderate hourly NO2 concentrations is likely to impact NO2AA at different types of sites across Europe. However, once emitted, NO2 has multiple other impacts. For example, NO2 is a key precursor for the formation of surface O3 (Karlsson et al., 2017; Monks et al., 2015), PAN (Fischer et al., 2014; Jenkin and Clemitshaw, 2000), secondary inorganic nitrate (Fuzzi et al., 2015; Putaud et al., 2010), and contributes to nitrogen deposition (Fowler et al., 2015; Galloway et al., 2008). To explore the conditions which result in the contribution of NO2 to these other impacts, an alternative set of chemical climate statistics would be required. Future work to derive and apply these statistics to the European monitoring network data could allow for similarity or differences in the conditions producing annual NO2 compared to the other above-mentioned impacts, and therefore facilitate a more comprehensive assessment of the effects of air pollution mitigation strategies. Co-located meteorological measurements with atmospheric composition measurements, as recommended by AQEG (2015), would also allow meteorological drivers of annual NO2 concentrations (and other impacts) to be assessed in more detail. Finally, the chemical climate statistics derived in this work have shown the variety of hourly NO2 contributions producing NO2AA across Europe. Application of these statistics to NO2 measurements from other regions could also facilitate comparison of the variation in monthly, hour of day, and hourly NO2 distributions that contribute to NO2AA in other regions.
An annual average limit value for NO2 concentrations for longer-term exposure of 40 µg m−3 has been established by the European Union for the protection of human health. Analysis of monitoring network measurements is key to assessing compliance with this limit value across Europe. The aim of this analysis was to link the magnitude of this impact metric to the monthly, hour of day, and hourly NO2 concentrations variation that produces it. Hence these contributions were calculated for 2010–2014 average annual NO2 concentrations for more than 2500 sites monitoring NO2 across Europe, and their trends estimated at 259 sites between 2000 and 2014.
This study shows that, in general, sites with distinct monthly, hour of day, and hourly NO2 concentration bin contributions to annual NO2 were not grouped into distinct geographic areas, and there were a range of patterns of hourly NO2 concentration variability producing similar annual NO2 concentrations within a particular area. This is a consequence of different interactions between emissions, atmospheric chemistry, and meteorology at different types of site. Hence within a city, country, or European region, there is variation in the extent to which annual NO2 concentrations could be reduced by focussing on, e.g. reducing peak NO2 concentrations compared to more moderate concentrations, winter vs. summer NO2 concentrations, and rush hour peaks compared to NO2 levels across the day. Therefore, the assessment of actions to mitigate annual average NO2 concentrations should evaluate their effect on hourly NO2 concentrations across the year, across the day, and across the hourly NO2 concentration distribution. Development of NO2 mitigation strategies should take into account that across Europe a variety of seasonal and diurnal patterns of hourly NO2 contribute to annual NO2 concentrations, and therefore that specific measures implemented to reduce NO2AA in one location may not be as effective in others.
The hourly NO2 measurement data used in this analysis were obtained from the publicly accessible European Environment Agency Airbase v8 (https://www.eea.europa.eu/data-and-maps/data/airbase-the-european-air-quality-database-8; EEA, 2017a) and Air Quality e-reporting (https://www.eea.europa.eu/data-and-maps/data/aqereporting-2; EEA, 2017b) data repositories.
The supplement related to this article is available online at: https://doi.org/10.5194/acp-18-3563-2018-supplement.
The authors declare that they have no conflict of interest.
The authors are grateful to Elizabeth Weatherhead for insightful discussions
regarding the trend analyses applied in this work. Sarah Moller acknowledges the
Natural Environment Research Council for funding (grant number
NE/N005430/1).
Edited by: Kyung-Eun Min
Reviewed by: two anonymous referees
Anenberg, S. C., Miller, J., Minjares, R., Du, L., Henze, D. K., Lacey, F., Malley, C. S., Emberson, L., Franco, V., Klimont, Z., and Heyes, C.: Impacts and mitigation of excess diesel-related NOx emissions in 11 major vehicle markets, Nature, 545, 467–471, 2017.
AQEG: Nitrogen Dioxide in the United Kingdom. Air Quality Expert Group Report, available at: https://uk-air.defra.gov.uk/library/aqeg/publications (last access: 8 March 2018), 2004.
AQEG: Trends in Primary Nitrogen Dioxide in the UK. Air Quality Expert Group Report, available at: https://uk-air.defra.gov.uk/assets/documents/reports/aqeg/primary-no-trends.pdf (last access: 8 March 2018), 2007.
AQEG: Evidential Value of Defra Air Quality Compliance Monitoring. Air Quality Expert Group, Defra Publications, available at: https://uk-air.defra.gov.uk/assets/documents/reports/cat11/1509290925_DEF-PB14312_Evidential_value_of_Defra_air_quality_compliance_monitoring.pdf (last access: 8 March 2018), 2015.
Austin, E., Coull, B., Zanobetti, A., and Koutrakis, P.: A framework to spatially cluster air pollution monitoring sites in US based on the PM2.5 composition, Environ. Int., 59, 244–254, 2013.
Baptista, P., Melo, S., and Rolim, C.: Energy, environmental and mobility impacts of car-sharing systems. Empirical results from Lisbon, Portugal, Procd. Soc. Behv., 111, 28–37, https://doi.org/10.1016/j.sbspro.2014.01.035, 2014.
Beevers, S. D., Westmoreland, E., de Jong, M. C., Williams, M. L., and Carslaw, D. C.: Trends in NOx and NO2 emissions from road traffic in Great Britain, Atmos. Environ., 54, 107–116, https://doi.org/10.1016/j.atmosenv.2012.02.028, 2012.
Blond, N., Boersma, K. F., Eskes, H. J., van der A, R. J., Van Roozendael, M., De Smedt, I., Bergametti, G., and Vautard, R.: Intercomparison of SCIAMACHY nitrogen dioxide observations, in situ measurements and air quality modeling results over Western Europe, J. Geophys. Res., 112, D10311, https://doi.org/10.1029/2006JD007277, 2007.
Box, G. E. and Jenkins, G. M.: Time Series Analysis, Control, and Forecasting, Holden Day, San Francisco, CA, USA, 1976.
Braniš, M.: Air quality of Prague: Traffic as a main pollution source, Environ. Monit. Assess., 156, 377–390, https://doi.org/10.1007/s10661-008-0491-3, 2009.
Carslaw, D. C. and Beevers, S. D.: Investigating the potential importance of primary NO2 emissions in a street canyon, Atmos. Environ., 38, 3585–3594, https://doi.org/10.1016/j.atmosenv.2004.03.041, 2004.
Carslaw, D. C. and Carslaw, N.: Detecting and characterising small changes in urban nitrogen dioxide concentrations, Atmos. Environ., 41, 4723–4733, https://doi.org/10.1016/j.atmosenv.2007.03.034, 2007.
Carslaw, D. C. and Ropkins, K.: openair – An R package for air quality data analysis, Environ. Modell. Softw., 27–28, 52–61, https://doi.org/10.1016/j.envsoft.2011.09.008, 2012.
Carslaw, D. C., Beevers, S., Westmoreland, E., Williams, W., Tate, J., Murrells, T., Stedman, J., Li, Y., Grice, S., Kent, A., and Tsagatakis, I.: Trends in NOx and NO2 emissions and ambient measurements in the UK, available at: https://uk-air.defra.gov.uk/assets/documents/reports/cat05/1108251149_110718_AQ0724_Final_report.pdf (last access: 8 March 2018), 2011.
Carslaw, D. C., Murrells, T. P., Andersson, J., and Keenan, M.: Have vehicle emissions of primary NO2 peaked?, Faraday Discuss., 189, 439–454, https://doi.org/10.1039/C5FD00162E, 2016.
Carugno, M., Consonni, D., Randi, G., Catelan, D., Grisotto, L., Bertazzi, P. A., Biggeri, A., and Baccini, M.: Air pollution exposure, cause-specific deaths and hospitalizations in a highly polluted italian region, Environ. Res., 147, 415–424, https://doi.org/10.1016/j.envres.2016.03.003, 2016.
Castellanos, P. and Boersma, K. F.: Reductions in nitrogen oxides over Europe driven by environmental policy and economic recession, Sci. Rep., 2, 265, https://doi.org/10.1038/srep00265, 2012.
CEBR: The future economic and environment costs of gridlock in 2030. Report for INRIX. Centre for Economics and Business Research. July 2014, available at: https://ibtta.org/sites/default/files/documents/MAF/Costs-of-Congestion-INRIX-Cebr-Report (3).pdf (last access: 8 March 2018), 2014.
Chaloulakou, A., Mavroidis, I., and Gavriil, I.: Compliance with the annual NO2 air quality standard in Athens. Required NOx levels and expected health implications, Atmos. Environ., 42, 454–465, https://doi.org/10.1016/j.atmosenv.2007.09.067, 2008.
Chiesa, M., Perrone, M. G., Cusumano, N., Ferrero, L., Sangiorgi, G., Bolzacchini, E., Lorenzoni, A., and Ballarin Denti, A.: An environmental, economical and socio-political analysis of a variety of urban air-pollution reduction policies for primary PM10 and NOx: The case study of the Province of Milan (Northern Italy), Environ. Sci. Policy, 44, 39–50, 2014.
Clapp, L. J. and Jenkin, M. E.: Analysis of the relationship between ambient levels of O3, NO2 and NO as a function of NOx in the UK, Atmos. Environ., 35, 6391–6405, https://doi.org/10.1016/S1352-2310(01)00378-8, 2001.
COMEAP: Interim Statement on Quantifying the Association of Long-Term Average Concentrations of Nitrogen Dioxide and Mortality. UK Comittee on the Medical Effects of Air Pollution, available at: https://www.gov.uk/government/uploads/system/uploads/attachment_data/file/485373/COMEAP_NO2_Mortality_Interim_Statement.pdf (last access: 8 March 2018), 2015.
Crouse, D. L., Peters, P. A., Hystad, P., Brook, J. R., van Donkelaar, A., Martin, R. V., Villeneuve, P. J., Jerrett, M., Goldberg, M. S., Pope C. Arden, I. I. I., Brauer, M., Brook, R. D., Robichaud, A., Menard, R., and Burnett, R. T.: Ambient PM2.5, O3, and NO2 Exposures and Associations with Mortality over 16 Years of Follow-Up in the Canadian Census Health and Environment Cohort (CanCHEC), Environ. Health Persp., 123, 1180–1186, https://doi.org/10.1289/ehp.1409276, 2015.
Cuevas, C. A., Notario, A., Adame, J. A., Hilboll, A., Richter, A., Burrows, J. P., and Saiz-Lopez, A.: Evolution of NO2 levels in Spain from 1996 to 2012, Sci. Rep., 4, 5887, https://doi.org/10.1038/srep05887, 2014.
Cyrys, J., Eeftens, M., Heinrich, J., Ampe, C., Armengaud, A., Beelen, R., Bellander, T., Beregszaszi, T., Birk, M., Cesaroni, G., Cirach, M., de Hoogh, K., De Nazelle, A., de Vocht, F., Declercq, C., Dedele, A., Dimakopoulou, K., Eriksen, K., Galassi, C., Graulevičiene, R., Grivas, G., Gruzieva, O., Gustafsson, A. H., Hoffmann, B., Iakovides, M., Ineichen, A., Krämer, U., Lanki, T., Lozano, P., Madsen, C., Meliefste, K., Modig, L., Mölter, A., Mosler, G., Nieuwenhuijsen, M., Nonnemacher, M., Oldenwening, M., Peters, A., Pontet, S., Probst-Hensch, N., Quass, U., Raaschou-Nielsen, O., Ranzi, A., Sugiri, D., Stephanou, E. G., Taimisto, P., Tsai, M. Y., Vaskövi, É., Villani, S., Wang, M., Brunekreef, B., and Hoek, G.: Variation of NO2 and NOx concentrations between and within 36 European study areas: Results from the ESCAPE study, Atmos. Environ., 62, 374–390, https://doi.org/10.1016/j.atmosenv.2012.07.080, 2012.
Donnelly, A., Misstear, B., and Broderick, B.: Application of nonparametric regression methods to study the relationship between NO2 concentrations and local wind direction and speed at background sites, Sci. Total Environ., 409, 1134–1144, https://doi.org/10.1016/j.scitotenv.2010.12.001, 2011.
Donnelly, A., Broderick, B., and Misstear, B.: Relating Background NO2 Concentrations in Air to Air Mass History Using Non-Parametric Regression Methods: Application at Two Background Sites in Ireland, Environ. Model. Assess., 17, 363–373, 2012.
EEA: Air Quality in Europe – 2016 report. European Environment Agency Report No. 28/2016, available at: http://www.eea.europa.eu/publications/air-quality-in-europe-2016 (last access: 8 March 2018), 2016a.
EEA: European Union emission inventory report 1990–2014 under the UNECE Convention on Long-range Transboundary Air Pollution (LRTAP), European Environment Agency Report No. 16/2016, Luxembourg, 2016b.
EEA: AirBase – The European air quality database v8, available at: https://www.eea.europa.eu/data-and-maps/data/airbase-the-european-air-quality-database-8 (last access: 8 March 2018), 2017a.
EEA: Air Quality e-Reporting (AQ e-Reporting), available at: https://www.eea.europa.eu/data-and-maps/data/aqereporting-2 (last access: 8 March 2018), 2017b.
European Commission: Guidance on the Commission Implementing Decision laying down rules for Directives 2004/107/EC and 2008/50/EC of the European Parliament and of the Council as regards the reciprocal exchange of information and reporting on ambient air, European Commission, Brussels, Belgium, 2013.
European Council Directive 2008/50/EC: On ambient air quality and cleaner air for Europe, 21 May 2008, L 152/1, Brussels, Belgium, 2008.
Fischer, E. V., Jacob, D. J., Yantosca, R. M., Sulprizio, M. P., Millet, D. B., Mao, J., Paulot, F., Singh, H. B., Roiger, A., Ries, L., Talbot, R. W., Dzepina, K., and Pandey Deolal, S.: Atmospheric peroxyacetyl nitrate (PAN): a global budget and source attribution, Atmos. Chem. Phys., 14, 2679–2698, https://doi.org/10.5194/acp-14-2679-2014, 2014.
Flemming, J., Stern, R., and Yamartino, R. J.: A new air quality regime classification scheme for O3, NO2, SO2 and PM10 observations sites, Atmos. Environ., 39, 6121–6129, 2005.
Fowler, D., Steadman, C. E., Stevenson, D., Coyle, M., Rees, R. M., Skiba, U. M., Sutton, M. A., Cape, J. N., Dore, A. J., Vieno, M., Simpson, D., Zaehle, S., Stocker, B. D., Rinaldi, M., Facchini, M. C., Flechard, C. R., Nemitz, E., Twigg, M., Erisman, J. W., Butterbach-Bahl, K., and Galloway, J. N.: Effects of global change during the 21st century on the nitrogen cycle, Atmos. Chem. Phys., 15, 13849–13893, https://doi.org/10.5194/acp-15-13849-2015, 2015.
Fuzzi, S., Baltensperger, U., Carslaw, K., Decesari, S., Denier van der Gon, H., Facchini, M. C., Fowler, D., Koren, I., Langford, B., Lohmann, U., Nemitz, E., Pandis, S., Riipinen, I., Rudich, Y., Schaap, M., Slowik, J. G., Spracklen, D. V., Vignati, E., Wild, M., Williams, M., and Gilardoni, S.: Particulate matter, air quality and climate: lessons learned and future needs, Atmos. Chem. Phys., 15, 8217–8299, https://doi.org/10.5194/acp-15-8217-2015, 2015.
Galloway, J. N., Townsend, A. R., Erisman, J. W., Bekunda, M., Cai, Z., Freney, J. R., Martinelli, L. A., Seitzinger, S. P., and Sutton, M. A.: Transformation of the Nitrogen Cycle: Recent Trends, Questions, and Potential Solutions, Science, 320, 889–892, https://doi.org/10.1126/science.1136674, 2008.
Geddes, J. A., Martin, R. V., Boys, B. L., and van Donkelaar, A.: Long-term trends worldwide in ambient NO2 concentrations inferred from satellite observations, Environ. Health Persp., 124, 281–289, https://doi.org/10.1289/ehp.1409567, 2016.
Gilge, S., Plass-Duelmer, C., Fricke, W., Kaiser, A., Ries, L., Buchmann, B., and Steinbacher, M.: Ozone, carbon monoxide and nitrogen oxides time series at four alpine GAW mountain stations in central Europe, Atmos. Chem. Phys., 10, 12295–12316, https://doi.org/10.5194/acp-10-12295-2010, 2010.
Grice, S., Stedman, J., Kent, A., Hobson, M., Norris, J., Abbott, J., and Cooke, S.: Recent trends and projections of primary NO2 emissions in Europe, Atmos. Environ., 43, 2154–2167, https://doi.org/10.1016/j.atmosenv.2009.01.019, 2009.
Hazenkamp-Von Arx, M. E., Götschi, T., Ackermann-Liebrich, U., Bono, R., Burney, P., Cyrys, J., Jarvis, D., Lillienberg, L., Luczynska, C., Maldonado, J. A., Jaén, A., De Marco, R., Mi, Y., Modig, L., Bayer-Oglesby, L., Payo, F., Soon, A., Sunyer, J., Villani, S., Weyler, J., and Künzli, N.: PM2.5 and NO2 assessment in 21 European study centres of ECRHS II: Annual means and seasonal differences, Atmos. Environ., 38, 1943–1953, https://doi.org/10.1016/j.atmosenv.2004.01.016, 2004.
Henschel, S., Le Tertre, A., Atkinson, R. W., Querol, X., Pandolfi, M., Zeka, A., Haluza, D., Analitis, A., Katsouyanni, K., Bouland, C., Pascal, M., Medina, S., and Goodman, P. G.: Trends of nitrogen oxides in ambient air in nine European cities between 1999 and 2010, Atmos. Environ., 117, 234–241, https://doi.org/10.1016/j.atmosenv.2015.07.013, 2016.
INRIX: London Congestion Trend. INRIX, available at: http://londonfirst.co.uk/wp-content/uploads/2016/05/London-Congestion-Trends-FINAL.pdf (last access: 8 March 2018), 2016.
Jeanjean, A. P. R., Gallagher, J., Monks, P. S., and Leigh, R. J.: Ranking current and prospective NO2 pollution mitigation strategies: An environmental and economic modelling investigation in Oxford Street, London, Environ. Pollut., 225, 587–597, https://doi.org/10.1016/j.envpol.2017.03.027, 2017.
Jenkin, M. E.: Investigation of the impact of short-timescale NOx variability on annual mean oxidant partitioning at UK sites, Atmos. Environ., 90, 43–50, https://doi.org/10.1016/j.atmosenv.2014.03.033, 2014.
Jenkin, M. E. and Clemitshaw, K. C.: Ozone and other secondary photochemical pollutants: chemical processes governing their formation in the planetary boundary layer, Atmos. Environ., 34, 2499–2527, 2000.
Joly, M. and Peuch, V. H.: Objective classification of air quality monitoring sites over Europe, Atmos. Environ., 47, 111–123, 2012.
JRC-AQUILA: JRC-AQUILA Position Paper: assessment on siting criteria, classification and representativeness of air quality monitoring stations, available at: http://ec.europa.eu/environment/air/pdf/SCREAM final.pdf (last access: 8 March 2018), 2013.
Karlsson, P. E., Klingberg, J., Engardt, M., Andersson, C., Langner, J., Karlsson, G. P., and Pleijel, H.: Past, present and future concentrations of ground-level ozone and potential impacts on ecosystems and human health in northern Europe, Sci. Total Environ., 576, 22–35, https://doi.org/10.1016/j.scitotenv.2016.10.061, 2017.
Kaufman, L. and Rousseeuw, P. J.: Finding Groups in Data: An Introduction to Cluster Analysis, Wiley, New York, USA, 1990.
Konovalov, I. B., Beekmann, M., Richter, A., Burrows, J. P., and Hilboll, A.: Multi-annual changes of NOx emissions in megacity regions: nonlinear trend analysis of satellite measurement based estimates, Atmos. Chem. Phys., 10, 8481–8498, https://doi.org/10.5194/acp-10-8481-2010, 2010.
Kunsch, H. R.: The Jackknife and the Bootstrap for General Stationary Observations, Ann. Stat., 17, 1217–1241, https://doi.org/10.1214/aos/1176347265, 1989.
Lozano, A., Usero, J., Vanderlinden, E., Raez, J., Contreras, J., and Navarrete, B.: Air quality monitoring network design to control nitrogen dioxide and ozone, applied in Malaga, Spain, Microchem. J., 93, 164–172, https://doi.org/10.1016/j.microc.2009.06.005, 2009.
Malley, C. S., Braban, C. F., and Heal, M. R.: New Directions: Chemical climatology and assessment of atmospheric composition impacts., Atmos. Environ., 87, 261–264, https://doi.org/10.1016/j.atmosenv.2014.01.027, 2014a.
Malley, C. S., Braban, C. F., and Heal, M. R.: The application of hierarchical cluster analysis and non-negative matrix factorization to European atmospheric monitoring site classification, Atmos. Res., 138, 30–40, 2014b.
Malley, C. S., Heal, M. R., Mills, G., and Braban, C. F.: Trends and drivers of ozone human health and vegetation impact metrics from UK EMEP supersite measurements (1990–2013), Atmos. Chem. Phys., 15, 4025–4042, https://doi.org/10.5194/acp-15-4025-2015, 2015.
Malley, C. S., Heal, M. R., Braban, C. F., Kentisbeer, J., Leeson, S. R., Lingard, J. J. N., Ritchie, S., Maggs, R., Beccaceci, S., Quincey, P., Brown, R. J. C., and Twigg, M. M.: The contributions to long-term health-relevant particulate matter at the UK EMEP supersites between 2010 and 2013: Quantifying the mitigation challenge, Environ. Int., 95, 98–111, 2016.
Mangiameli, P., Chen, S. K., and West, D.: A comparison of SOM neural network and hierarchical clustering methods, Eur. J. Oper. Res., 93, 402–417, 1996.
Mann, H. B.: Nonparametric tests against trend, Econometrica, 13, 245–259, 1945.
Masiol, M., Squizzato, S., Formenton, G., Harrison, R. M., and Agostinelli, C.: Air quality across a European hotspot: Spatial gradients, seasonality, diurnal cycles and trends in the Veneto region, NE Italy, Sci. Total Environ., 576, 210–224, https://doi.org/10.1016/j.scitotenv.2016.10.042, 2017.
Mavroidis, I. and Chaloulakou, A.: Long-term trends of primary and secondary NO2 production in the Athens area. Variation of the NO2 ∕ NOx ratio, Atmos. Environ., 45, 6872–6879, https://doi.org/10.1016/j.atmosenv.2010.11.006, 2011.
Mavroidis, I. and Ilia, M.: Trends of NOx, NO2 and O3 concentrations at three different types of air quality monitoring stations in Athens, Greece, Atmos. Environ., 63, 135–147, https://doi.org/10.1016/j.atmosenv.2012.09.030, 2012.
Monks, P. S., Archibald, A. T., Colette, A., Cooper, O., Coyle, M., Derwent, R., Fowler, D., Granier, C., Law, K. S., Mills, G. E., Stevenson, D. S., Tarasova, O., Thouret, V., von Schneidemesser, E., Sommariva, R., Wild, O., and Williams, M. L.: Tropospheric ozone and its precursors from the urban to the global scale from air quality to short-lived climate forcer, Atmos. Chem. Phys., 15, 8889–8973, https://doi.org/10.5194/acp-15-8889-2015, 2015.
Palarz, A., Celinski-Myslaw, D., and Ustrnul, Z.: Temporal and spatial variability of surface-based inversions over Europe based on ERA-Interim reanalysis, Int. J. Climatol., 38, 158–168, https://doi.org/10.1002/joc.5167, 2017.
Putaud, J. P., Van Dingenen, R., Alastuey, A., Bauer, H., Birmili, W., Cyrys, J., Flentje, H., Fuzzi, S., Gehrig, R., Hansson, H. C., Harrison, R. M., Herrmann, H., Hitzenberger, R., Hüglin, C., Jones, A. M., Kasper-Giebl, A., Kiss, G., Kousa, A., Kuhlbusch, T. A. J., Löschau, G., Maenhaut, W., Molnar, A., Moreno, T., Pekkanen, J., Perrino, C., Pitz, M., Puxbaum, H., Querol, X., Rodriguez, S., Salma, I., Schwarz, J., Smolik, J., Schneider, J., Spindler, G., ten Brink, H., Tursic, J., Viana, M., Wiedensohler, A., and Raes, F.: A European aerosol phenomenology – 3: Physical and chemical characteristics of particulate matter from 60 rural, urban, and kerbside sites across Europe, Atmos. Environ., 44, 1308–1320, https://doi.org/10.1016/j.atmosenv.2009.12.011, 2010.
R Core Team: R: a Language and Environment for Statistical Computing. R Foundation for Statistical Computing, Vienna, Austria, available at: https://www.R-project.org/ (last access: 8 March 2018), 2016.
Reed, C., Evans, M. J., Di Carlo, P., Lee, J. D., and Carpenter, L. J.: Interferences in photolytic NO2 measurements: explanation for an apparent missing oxidant?, Atmos. Chem. Phys., 16, 4707–4724, https://doi.org/10.5194/acp-16-4707-2016, 2016.
REVIHAAP: Review of evidence on health aspects of air pollution – REVIHAAP Project technical report. World Health Organization (WHO) Regional Office for Europe, Bonn, Germany, available: http://www.euro.who.int/__data/assets/pdf_file/0004/193108/REVIHAAP-Final-technical-report-final-version.pdf?ua=1 (last access: 8 March 2018), 2013.
Schäfer, K., Emeis, S., Hoffmann, H., and Jahn, C.: Influence of mixing layer height upon air pollution in urban and sub-urban areas, Meteorol. Z., 15, 647–658, https://doi.org/10.1127/0941-2948/2006/0164, 2006.
Schneider, P., Lahoz, W. A., and van der A, R.: Recent satellite-based trends of tropospheric nitrogen dioxide over large urban agglomerations worldwide, Atmos. Chem. Phys., 15, 1205–1220, https://doi.org/10.5194/acp-15-1205-2015, 2015.
Seidel, D. J., Zhang, Y., Beljaars, A., Golaz, J.-C., Jacobson, A. R., and Medeiros, B.: Climatology of the planetary boundary layer over the continental United States and Europe, J. Geophys. Res.-Atmos., 117, D17106, https://doi.org/10.1029/2012JD018143, 2012.
Spangl, W., Schneider, J., Moosmann, L., and Nagi, C.: Representativeness and Classification of Air Quality Monitoring Stations, Umweltbundesamt Report, available at: http://www.umweltbundesamt.at/fileadmin/site/publikationen/REP0121.pdf (last access: 8 March 2018), 2007.
Stathopoulos, A. and Karlaftis, M.: Temporal and spatial variations of real-time traffic data in urban areas, Trans. Res. B., 1768, 135–140, https://doi.org/10.3141/1768-16, 2001.
Tarasova, O. A., Brenninkmeijer, C. A. M., Jöckel, P., Zvyagintsev, A. M., and Kuznetsov, G. I.: A climatology of surface ozone in the extra tropics: cluster analysis of observations and model results, Atmos. Chem. Phys., 7, 6099–6117, https://doi.org/10.5194/acp-7-6099-2007, 2007.
Theil, H.: A rank-invariant method of linear and polynomial regression analysis I, Proc. Kon. Ned. Akad. V. Wetensch. A, 53, 386–392, 1950a.
Theil, H.: A rank-invariant method of linear and polynomial regression analysis II, Proc. Kon. Ned. Akad. V. Wetensch. A, 53, 521–525, 1950b.
Theil, H.: A rank-invariant method of linear and polynomial regression analysis III, Proc. Kon. Ned. Akad. V. Wetensch. A, 53, 1397–1412, 1950c.
Thomas, M.: Research for TRAN committee – The World is Changing. Transport Too. Policy Department B: Structural and Cohesion Policies, European Parliament, available at: http://www.europarl.europa.eu/RegData/etudes/STUD/2016/563424/IPOL_STU(2016)563424_EN.pdf (last access: 8 March 2018), 2016.
Thomas, T., Weijermars, W., and van Berkum, E.: Variations in urban traffic volumes, Eur. J. Transp. Infrast., 8, 251–263, 2008.
Turner, M. C., Jerrett, M., Pope III, C. A., Krewski, D., Gapstur, S. M., Diver, W. R., Beckerman, B. S., Marshall, J. D., Su, J., Crouse, D. L., and Burnett, R. T.: Long-Term Ozone Exposure and Mortality in a Large Prospective Study, Am. J. Resp. Crit. Care, 193, 1134–1142, https://doi.org/10.1164/rccm.201508-1633OC, 2016.
US EPA: Integrated Science Assessment for Oxides of Nitrogen – Health Criteria. United States Environmental Protection Agency Report Number EPA/600/R-15/068, available at: https://www.epa.gov/isa/integrated-science-assessment-isa-nitrogen-dioxide-health-criteria (last access: 8 March 2018), 2016.
Vestreng, V., Ntziachristos, L., Semb, A., Reis, S., Isaksen, I. S. A., and Tarrasón, L.: Evolution of NOx emissions in Europe with focus on road transport control measures, Atmos. Chem. Phys., 9, 1503–1520, https://doi.org/10.5194/acp-9-1503-2009, 2009.
Villena, G., Bejan, I., Kurtenbach, R., Wiesen, P., and Kleffmann, J.: Interferences of commercial NO2 instruments in the urban atmosphere and in a smog chamber, Atmos. Meas. Tech., 5, 149–159, https://doi.org/10.5194/amt-5-149-2012, 2012.
Wagner, P. and Schäfer, K.: Influence of mixing layer height on air pollutant concentrations in an urban street canyon, Urban Clim., 22, 1–13, https://doi.org/10.1016/j.uclim.2015.11.001, 2015.
Ward, J.: Hierarchical Grouping to Optimize an Objective Function, J. Am. Stat. Assoc., 58, 236–244, 1963.
Weijermars, W.: Analysis of urban traffic patterns using clustering. TRAIL Thesis Series T2007/3, The Netherlands TRAIL Research School, PhD Thesis, University of Twente, available at: https://ris.utwente.nl/ws/portalfiles/portal/6069766 (last access: 8 March 2018), 2007.
WHO: Air quality guidelines: Global update 2005. Particulate matter, ozone, nitrogen dioxide and sulfur dioxide. World health organization regional office for europe, available at: http://www.euro.who.int/__data/assets/pdf_file/0005/78638/E90038.pdf?ua=1 (last access: 8 March 2018), 2006.
Zhang, Y., Seidel, D. J., and Zhang, S.: Trends in planetary boundary layer height over europe, J. Climate, 26, 10071–10076, https://doi.org/10.1175/JCLI-D-13-00108.1, 2013.