the Creative Commons Attribution 4.0 License.
the Creative Commons Attribution 4.0 License.
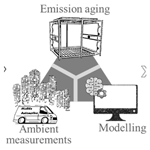
Opinion: How will advances in aerosol science inform our understanding of the health impacts of outdoor particulate pollution?
Danielle Vienneau
Kaspar R. Daellenbach
Robin Modini
Jay G. Slowik
Abhishek Upadhyay
Petros N. Vasilakos
David Bell
Kees de Hoogh
Andre S. H. Prevot
Air pollution, characterized by high levels of particulate matter (PM), poses the greatest environmental threat to human health, causing an estimated 7 million deaths annually and accounting for 5 % of the global gross domestic product (GDP). While the health impacts of PM are influenced by the toxicity of its individual chemical constituents, the mortality burden of PM is solely based on its total mass concentration. This is because of a lack of large-scale, high-resolution data on PM chemical composition, needed for epidemiological assessments. Identifying which PM constituents are harmful to health has been the “holy grail” of atmospheric science since the landmark 1993 study on six US cities established a definitive link between PM and mortality. Ever since, atmospheric scientists have focused on understanding aerosol composition, emission sources, and formation pathways, while longitudinal epidemiological studies have required individual-level exposure data, employing land use regression models for the prediction of exposures at fine resolutions. In this opinion article, we argue that the time has come to shift the focus towards incorporating PM chemical composition into epidemiological health assessments, laying the foundation for the development of new regulatory metrics. This shift will enable the creation of targeted guidelines and subsequent regulations, prioritizing mitigation efforts against the most harmful anthropogenic emissions. Central to this shift is the availability of global, long-term, high-resolution data on PM chemical composition that are obtained through field observations and modelling outputs. In the article, we underscore key milestones within aerosol science that have been integral for advancing this foundational shift. Specifically, we examine emerging modelling tools for estimating exposure to individual PM components, present the type of ambient observations needed for model developments, identify key gaps in our fundamental understanding of emissions and their atmospheric transformation, and propose advancing cross-disciplinary collaboration between aerosol scientists and epidemiologists to understand the health impacts of individual PM components. We contend that aerosol science has now reached a pivotal moment in elucidating the differential health impacts of PM components, representing a first step towards their incorporation into air quality guidelines.
- Article
(2166 KB) - Full-text XML
- BibTeX
- EndNote
1.1 A brief chronology of air pollution
A tale of global air pollution has already been provided by Fowler et al.; thus, only a brief chronology follows, presenting the main milestones reached by the atmospheric-science community since the earliest recorded accounts of air pollution (Fowler et al., 2020). The threat of air pollution to human health has been recognized since the time of Hippocrates, around 400 BCE (Jones et al., 1923). Written accounts of air pollution emerged throughout the following 2 millennia until measurements from the 18th century onwards demonstrated the growing scale of poor air quality in urban centres. One of the most emblematic early historical documents on air pollution was published by Evelyn (1772) in 1661 under the title Fumifugium. Evelyn documented air pollution in London and proposed solutions for reducing the scale of the problem, such as moving industries to the countryside. Graunt, a contemporary of Evelyn, observed a correlation between rates of mortality and pollution, especially during fog episodes, despite lacking any chemical data or numerical values for quantifying the pollutants present (Graunt, 1939). Later, in 1775, Sir Percival Pott was one of the first to document the effects of specific pollutants on health. Pott observed a high incidence of scrotal cancer among chimney sweeps and concluded that exposure to soot was a risk factor for this type of cancer (Brown and Thornton, 1957).
The Industrial Revolution accelerated the growth and geographical spread of emissions, leading to the defining problem of highly polluted cities, which culminated in the Great Smog of London in 1952. This pollution episode, lasting a few days, caused an estimated 10 000 deaths and injury to more than 100 000 people (Stone, 2002; Bell et al., 2004). The Great Smog of London is believed to be the worst air pollution event in the history of the United Kingdom and the most notorious air pollution event due to its effects on environmental research, government regulation, and public awareness of the relationship between air quality and health. It was instrumental in establishing an unambiguous link between short-term exposure to peak levels of pollution and acute health effects. It also led to the introduction of the 1956 Clean Air Act, which aimed to reduce emissions and mitigate future pollution events. Until the latter decades of the 20th century, Europe and North America were the primary sources of global emissions and experienced most of the resulting adverse health and environmental effects. By that time, transboundary issues concerning acid rain (Egnér and Eriksson, 1955) and ground-level ozone (Volz and Kley, 1988; Fowler et al., 2008) had become major environmental and political air quality problems (Vasseur, 1973). As emission controls took effect in the western world, Asia rapidly industrialized, emerging as the primary contributor to global emissions by the early 21st century.
Towards the end of the 20th century, the health effects of air pollution resurfaced as a top priority, with new epidemiological evidence highlighting the breadth of chronic health problems resulting from long-term pollution exposure (Brunekreef and Holgate, 2002). To address this, the emergence of extensive networks of surface measurements, satellite remote sensing, and numerical models was indispensable, providing global air quality data that enabled epidemiologists to estimate the adverse health effects of air pollution. Since then, numerous studies have documented the chronic and acute health effects of air pollution and the associated burden of disease, many of which provide a global perspective (Burnett et al., 2014, 2018; Cohen et al., 2017; McDuffie et al., 2021; Lelieveld et al., 2015, 2019; Chen et al., 2018b; Chen and Hoek, 2020; de Bont et al., 2022; Holtjer et al., 2023; Nyadanu et al., 2022). Today, air pollution remains a major public-health concern, and efforts to reduce emissions and improve air quality are ongoing.
1.2 Particulate air pollution
The polluted air we breathe can contain high levels of particulate matter (PM), commonly referred to as aerosols. PM is a complex mixture of tiny solid or liquid particles suspended in the air, ranging in size from a few nanometres to a few micrometres (Seinfeld and Pandis, 2016). These particles can be directly emitted from primary sources or formed in the atmosphere through gas-to-particle conversion of secondary oxidation products (e.g. sulfate from SO2 oxidation, nitrate from NOX oxidation, or secondary organic aerosols (SOAs) from the oxidation of volatile organic vapours). Primary PM sources can be either natural or human-made. Natural sources include desert dust, sea spray, wildfires, and biogenic SOAs from the oxidation of plant volatiles, while anthropogenic sources include emissions from residential heating or car exhausts and their secondary oxidation products. As a result, PM has an immensely complex chemical composition, exhibiting different levels of toxicity depending on the emission sources and/or formation processes (Hallquist et al., 2009; Jimenez et al., 2009). Smaller particles are more likely to enter our bloodstream and travel deep into our lungs, causing damage. Short-term exposure to peak levels of PM can cause acute health effects (Dockery et al., 1993; Liu et al., 2023; Nhung et al., 2017). By contrast, long-term exposure can lead to chronic diseases, such as cardiovascular, cerebrovascular (de Bont et al., 2022), and respiratory diseases (Holtjer et al., 2023), which are responsible for a large share of air-pollution-related mortality (Burnett et al., 2018; Cohen et al., 2017; Chen and Hoek, 2020). Current epidemiological evidence reveals that no level of air pollution can be deemed safe, and even low levels of PM may carry significant risks (Strak et al., 2021; Pinault et al., 2016; Cohen et al., 2017; Dominici et al., 2022; Brunekreef, 2021; Brauer et al., 2019). Today, according to the Global Burden of Disease study, PM pollution is responsible for an estimated 7 million deaths each year (Burnett et al., 2018). This positions PM among the five leading causes of death worldwide, alongside high blood pressure, smoking, diabetes, and obesity (Cohen et al., 2017).
1.3 PM mitigation: a global challenge of the 21st century
Although particles are compositionally heterogeneous, exhibiting notable temporal and spatial variations, most studies investigating their adverse health effects have used total PM mass concentration, treating particles as a uniform entity. This is because total PM mass concentration has been routinely measured, primarily as PM2.5 in the USA and PM10 in Europe1, and used – either directly or in the form of models – as the basis of exposure assessments in epidemiological studies. As a result, based on the accumulated evidence, PM mass serves today as the primary metric for particulate pollution regulation.
In 2021, the World Health Organization (WHO) updated its air quality guidelines to propose a much more stringent limit value for PM2.5 of 5 µg m−3 (WHO, 2021). These new guidelines provide a basis for justifying the aggressive regulation of anthropogenic emissions to improve global air quality. However, PM2.5 concentrations this low are currently only found in a few remote environments, and over 95 % of the world's population lives in places where the new guidelines are not met. Several western countries have made significant progress over the past 20 years in meeting the former WHO limit for PM2.5 of 10 µg m−3, which was last updated in 2005 (Southerland et al., 2022; Hammer et al., 2020). In contrast, PM2.5 levels exceeding 50 µg m−3 are typical in low- to middle-income countries (e.g. countries in Eastern Europe, China or India), where 90 % of attributable deaths occur (Lelieveld et al., 2015). This translates to a loss of several years of life expectancy in Asia due to pollution, whereas this loss is limited to a few months in the western world (Lelieveld et al., 2019).
Reducing fossil fuel dependence and residential emissions will undoubtedly significantly improve air quality, especially in polluted environments (Pai et al., 2022; McDuffie et al., 2021). However, natural sources, including desert dust, wildfires, and biogenic emissions, will continue to impede many regions from complying with the new WHO guidelines. This, however, is not a reason to dismiss the evidence-based limit values set by the WHO in 2021, which are intended to protect health; instead, it is a reason to further improve them. A recent landmark modelling analysis suggested that over 50 % of the global population will still live in areas with PM2.5 concentrations greater than 5 µg m−3, even if all anthropogenic emissions were eliminated (Pai et al., 2022). Moreover, natural emissions are likely to increase in the near future due to climate change, further complicating efforts to meet the 2021 WHO guidelines in certain regions (Gomez et al., 2023). Meeting these guidelines will be particularly challenging for many regions worldwide, and globally applicable solutions for managing and improving air quality will become increasingly elusive. This necessitates a complete rethink of how we should mitigate air pollution and underlines the need for a new generation of air quality metrics that focus on specific anthropogenic PM components in addition to total PM mass.
Targeting particulate pollution across individual chemical components allows for the consideration of their varying degrees of toxicity, a concept known as the “differential toxicity of PM components” (Masselot et al., 2022). PM health effects are mediated by the size, solubility, and chemical composition of PM, and, hence, by its sources and formation processes. In our recent work, we identified organic and metal fractions as being of particular concern for oxidative stress (Daellenbach et al., 2020) and inflammation (Leni et al., 2020), contrasting with secondary inorganic particles that dominate PM mass. Given that oxidative stress and inflammation are pathways to the development of chronic diseases (Mudway et al., 2020), a closer inspection of which PM sources should be mitigated is required. It is therefore vitally important for aerosol scientists to provide global data on PM chemical composition, enabling epidemiologists to pinpoint the most detrimental components and empowering policymakers to implement targeted, cost-effective measures to reduce specific health-relevant, anthropogenic PM sources across different regions.
1.4 Introductory overview
In this account, we discuss how the broader atmospheric-science community can inform policies and interventions to mitigate sources of PM components that pose risks to human health (Fig. 1). We advocate for a foundational shift towards considering PM deferential toxicity in epidemiological health assessments, made possible through improved air quality modelling suitable for exposure assessment, and present the key milestones within aerosol science that, in our view, are necessary for this shift. Section 2 introduces the concept of PM differential toxicity and its potential as an exposure metric. Section 3 critically examines recent advances in modelling tools for estimating fine-scale exposures to specific PM components. In Sect. 4, we identify the types of ambient observations we consider essential for developing and validating exposure models. In Sect. 5, we highlight remaining gaps in our understanding of PM component emissions, their atmospheric transformation, and associated health effects, before identifying research opportunities. Section 6 delves into the need for strong collaboration between research communities to elucidate the biological mechanisms underlying the health impacts of specific PM components.
2.1 PM differential toxicity as a targeted air quality metric: more than just PM mass
To quantify the health impacts of PM, we currently rely on dose–response relationships (ideally derived from individual-level data from large cohort studies) that link morbidity and mortality to the concentration of total PM mass. While these relationships are based on a broad evidence base, there is significant heterogeneity in the estimated effect size. This variation can be partially attributed to imperfect models approximating exposure or random differences among study populations. However, a large source of error may lie in relying solely on PM mass concentration, thereby ignoring the biological activity of different particle constituents. Although some studies have attempted to examine the adverse health outcomes of individual PM components, particularly highlighting associations with combustion and road traffic emissions, such investigations remain relatively infrequent due to the paucity of exposure data. As a result, PM chemical composition has not yet been integrated into any regulatory framework.
With the advent of vast amounts of atmospheric data, the time has now come to redirect our focus towards elucidating dose–response relationships for individual PM constituents. In practical terms, these constituents must be quantifiable, easily accessible, and readily available at the high spatiotemporal resolutions needed for exposure assessments and across large spatial scales. Our proposal includes considering the following constituents: organic aerosols, elemental carbon, sulfate, nitrate, ammonium, sea salt, brake wear, and dust (Table 1). While brake wear and dust concentrations cannot be directly measured, they can be traced using specific markers, such as Cu for brake wear and Al for dust. The organic fraction should ideally be subdivided based on source sectors, including primary and secondary aerosols from car exhausts, residential burning, wildfires, and biogenic emissions. Classes of organic aerosols also cannot be directly measured, although they can be retrieved through receptor modelling based on spectrometric measurements or chemical transport modelling, as discussed below. The classification of aerosols based on their chemical composition also establishes a direct link to aerosol sources (Table 1), offering policymakers effective and operational strategies for selectively mitigating the most important PM sources for health.
Beyond PM chemical composition, various other properties have been proposed to mediate aerosol health effects, including aerosol size, number, solubility, and oxidative potential (Table 1). For example, toxic metals primarily induce oxidative damage in their soluble form (Fang et al., 2017; Wong et al., 2020), whereas insoluble particles (such as asbestos or elemental carbon) are biopersistent in the body, leading to chronic inflammation. Likewise, small particles can penetrate deep into the lungs, traverse the bloodstream, and breach the blood–brain barrier, playing a role in respiratory, cardiovascular, and neurological diseases (Requia et al., 2017; Maher et al., 2016), whereas a notable portion of large particles may be ingested, leading to an imbalance in our gut microbiome (Fouladi et al., 2020; Alderete et al., 2018; Bailey et al., 2020). Parameters for emerging metrics intended for use in future epidemiological studies should be standardized and widely available, which is currently unachievable for some of these parameters. PM chemical composition, which is now widely available, is intertwined with these alternative metrics (Table 1) and can serve as an effective means of addressing PM health effects.
2.2 Necessity of data on PM chemical composition at fine resolutions
More than 70 % of the world population is projected to live in urban areas by 2050, with these densely populated agglomerations already being the most polluted environments (McDuffie et al., 2021). The composition and concentrations of PM in these areas exhibit significant spatial heterogeneity from street to city-wide scales. In some cases, intra-city variability exceeds the variability between different cities (de Hoogh et al., 2016, 2013; Eeftens et al., 2016, 2012s; Tsai et al., 2015; Zhang et al., 2015; Jedynska et al., 2015). Such spatial heterogeneity is driven by traffic patterns (Simon et al., 2017; H. Z. Li et al., 2016; Gu et al., 2018; Elser et al., 2018, 2016), restaurant emissions (Gu et al., 2018), domestic-heating emissions (Elser et al., 2018, 2016; Jedynska et al., 2015; Mohr et al., 2011), industrial point sources (Shairsingh et al., 2018), and local geography (Mohr et al., 2011). Atmospheric ageing of urban emissions and long-range transport of polluted air masses add to this complexity, affecting PM background levels and composition on regional scales.
Table 1This table displays the chemical components of PM, reflecting their differential toxicity, which should be monitored and modelled to support health research. The physical properties of these components that serve as important determinants of health effects are shown; these include size, morphology, and solubility. The components' major sources are also shown. (a, b, c) Images derived from transmission electron microscopy of organic aerosols, elemental-carbon aggregates, and sulfate, adapted from Li et al. (2011). (d) Images derived from scanning electron microscopy of a fresh sea salt particle, adapted from W. Li et al. (2016). (e) Scanning electron microscopic image of a coarse brake wear particle, adapted from Kukutschová and Filip (2018). (f) Images derived from transmission electron microscopy of a mineral dust particle, adapted from Xu et al. (2021).
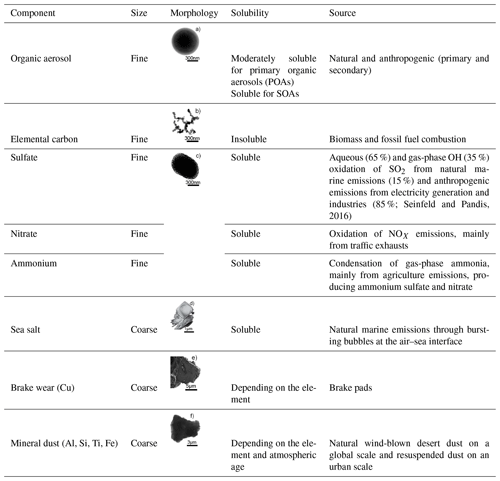
Urban microenvironments strongly affect long-term exposures to specific PM components (Fig. 2a). For example, studies have shown a link between road proximity; exposure to ultrafine particles; and respiratory, cardiovascular, and neurodegenerative diseases (Alexeeff et al., 2018; Bayer-Oglesby et al., 2006; Yuchi et al., 2020; Boogaard et al., 2022). It has also been shown that exposure to high particle concentrations around train stations during typical daily commutes of less than 1 hour can account for up to 21 % of total daily PM exposure and more than 50 % of daily exposure to key toxic metals, e.g. Cu (Van Ryswyk et al., 2017). Given the substantial spatiotemporal variability in both PM concentrations and composition, it is imperative to determine PM chemical composition at fine spatial and temporal scales that are relevant to daily human activities. This understanding is vital for evaluating exposures to specific PM components and, ultimately, comprehending their health effects.
In epidemiological analyses, outdoor PM concentrations at residences are commonly used as proxies for exposure. While there is evidence supporting this approach, the applicability of this approach across different settings requires further investigation (Wei et al., 2023). As we spend most of our time indoors and new buildings are increasingly airtight for energy saving, outdoor concentrations may not reflect indoor levels (Schweizer et al., 2007). While indoor emissions, primarily from cooking (Klein et al., 2019) and smoking (Hyland et al., 2008), may influence health, they represent a separate risk factor that is distinct from outdoor air pollution, similar to the issue of contaminated water. This is because (1) different regulatory frameworks are needed to address emissions from indoor and outdoor sources, (2) these sources are often distinct, and (3) they require different control measures. Unlike outdoor air pollution, which often requires collective and regulatory abatement strategies to control emissions, indoor air pollution can be more effectively managed at the individual or household level by improving ventilation and eliminating or reducing indoor sources. In the absence of indoor emissions, indoor concentrations are 30 % to 70 % lower than outdoor levels (Chen and Zhao, 2011) due to variability in infiltration rates. Moreover, exposure can be influenced by outdoor pollution in other settings, such as in workplaces and during commuting, where we spend a large fraction of our time. Health data from citizen cohorts often include questionnaires that offer valuable insights into indoor infiltration rates, workplace conditions, and individual mobility. While we consider outdoor concentrations at residences to be a reasonable proxy for exposure to outdoor pollution, integrating such information can help refine exposure estimations. However, before this can be resolved, the issue of downscaling air quality models to finer resolutions must be tackled.
Investigating acute health effects requires analysing time series of daily exposure to specific components, typically obtained through long-term measurements (> 3 years) at urban background sites or from modelling outputs. In contrast, longitudinal epidemiological studies of chronic diseases require long-term exposure data at a high spatial resolution – ideally at the address level. Because high-resolution PM composition data are scarce, existing epidemiological analyses considering PM chemical composition have mainly focused on acute effects, whereas health effects resulting from chronic exposure to individual PM components have rarely been assessed. In this section, we define the state-of-the-art method for modelling PM exposure and discuss how recent advances in modelling PM chemical composition can help inform our understanding of PM differential toxicity.
3.1 Existing modelling approaches
Figure 2b compares three traditional approaches for estimating PM exposures. We propose eight criteria for comparing these approaches: accuracy, spatial and temporal resolution, spatial and temporal coverage, capability for hindcasting and forecasting (i.e. estimating past and future exposures), source specificity, and chemical complexity (i.e. the ability to quantify specific PM components).
Early cohort studies used averaged (Pope III et al., 2002) or interpolated (Jerrett et al., 2005) PM concentrations measured at routine monitoring stations to characterize the exposure of individual participants across different cities. However, stationary PM measurements are spatially sparse and do not account for the heterogeneity in pollutant concentrations within cities, especially with regard to primary combustion emissions (Eeftens et al., 2012b; Elser et al., 2016, 2018). Therefore, several geostatistical and process-based chemical transport models have been proposed to fill these spatial gaps.
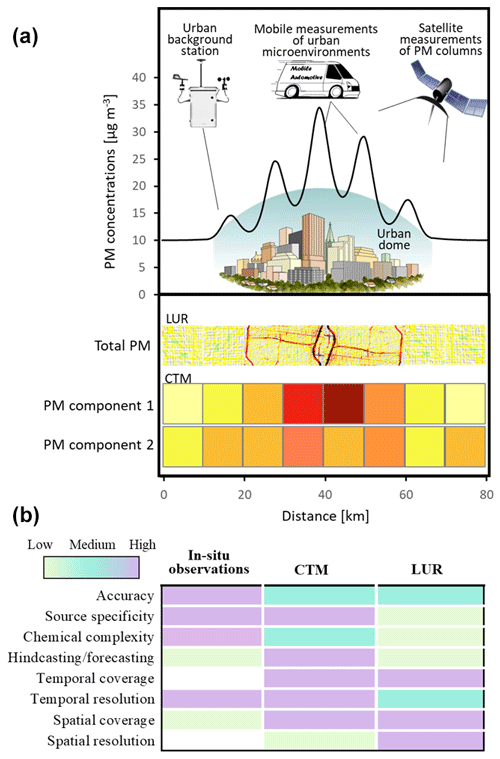
Figure 2(a) Representation of urban PM pollution, highlighting the urban increments in PM concentrations over background levels and the presence of microenvironments. State-of-the-art measurement and modelling strategies for PM concentrations at different scales are presented and compared in panel (b) in terms of their advantages and limitations. Three different approaches are compared: field observations, chemical transport models (CTMs), and land use regression (LUR) models. The temporal coverage and spatial resolution of in situ observations are determined by the method employed to obtain them; white cells correspond to non-applicability. A comparison of the performance of CTMs vs. LUR models is illustrated in panel (a), showing the source specificity of CTMs and the high resolution of LUR models.
Land use regression (LUR) models combine in situ observations with data based on Geographic Information Systems (GISs; e.g. data on land use, traffic, or population density) as emission indicators to predict ground-level PM concentrations on fine grids using regression techniques (Cattani et al., 2017; de Hoogh et al., 2016, 2013; Eeftens et al., 2016; Hoek et al., 2011; Kim et al., 2016; Wolf et al., 2017). These techniques are covered in a review by Hoek (2017). LUR techniques are especially useful for modelling primary PM components, e.g. metals (Kim et al., 2016; J. Chen et al., 2020) and combustion products (Jedynska et al., 2014, 2015), as well as PM mass (Eeftens et al., 2012a). Typically, these LUR models predict annual means, which are suitable for longitudinal cohort studies on chronic health effects. Their accuracy is moderate and influenced by the number of monitoring sites (Wang et al., 2013) and the inclusion of source-specific predictor variables.
With advances in satellite remote sensing, aerosol-optical-depth (AOD) measurements of entire atmospheric columns now provide a much more accurate assessment of exposure to ground-level total PM mass at a relatively high time resolution. Because AOD–PM relationships are non-linear, interactive, and spatiotemporally variable, AOD measurements are typically combined with other predictors, including land use data and meteorological variables. Machine-learning-based models using geostatistical and AOD data have been successfully applied at different scales, including city, regional, national, and continental scales, as well as in different areas around the world, including the EU, the US, and China (Brokamp et al., 2017; Suleiman et al., 2016; Huang et al., 2018; de Hoogh et al., 2018; Di et al., 2016; Hu et al., 2017; Paciorek et al., 2008; Strawa et al., 2013; Zhan et al., 2017; Di et al., 2019; Xue et al., 2019; J. Chen et al., 2018). However, because they are based on past AOD measurements, these models cannot forecast future PM concentrations, such as those responding to specific mitigation strategies (Fig. 2b). More importantly, they are typically not capable of differentiating between specific PM components because AOD measurements of PM columns are not yet chemically resolved, although future satellite-based sensors will partially provide this capability (David et al., 2018).
Unlike other methods, CTMs possess the ability to generate spatial and temporal distributions of chemically resolved PM components and forecast their future evolutions over large spatial scales. CTMs involve bottom-up, process-based numerical models which simulate PM primary emissions and secondary formation, along with their losses and atmospheric transport, in large 3D gridded domains. Despite their spatial coverage, source specificity, and ability to leverage complex atmospheric oxidation processes, most CTMs lack the spatial resolution needed to be suitable for exposure assessments (Fig. 2b). Due to computational constraints, highly resolved CTMs are currently limited to city scales. As a result, until very recently, CTM outputs have rarely been exploited for epidemiological analysis, except in the form of input variables for optimizing the retrieval of total PM mass concentrations in hybrid LUR models (Di et al., 2019; Xue et al., 2019; de Hoogh et al., 2016; Shen et al., 2022).
These two fields of air quality modelling, i.e. using LUR models and CTMs, have evolved along separate trajectories over the past 3 decades. This separation can be attributed to the modest accuracy of CTMs in the past and their coarse spatial resolution, which struggled to represent the substantial contribution of local pollution. Epidemiologists later applied LUR techniques, taking advantage of their high spatial resolution, reliance on locally measured air pollution data, ease of application, minimal input data requirements, and moderate to good performance (Hoek, 2017). At that time, CTM developments primarily focused on implementing representative emission and chemical schemes to enhance their accuracy. However, with advancements in CTMs and the increasing regional nature of PM pollution, it is now time for these two fields to converge to achieve an accurate estimation of exposure to individual PM components at high temporal and spatial resolutions and with high coverage, fulfilling all eight criteria described in Fig. 2b.
3.2 How can recent advances in modelling PM chemical composition help inform our understanding of PM differential toxicity?
Recent modelling developments have enabled the production of fine-resolution maps of PM chemical constituents on continental and global scales (Van Donkelaar et al., 2019; J. Chen et al., 2020; McDuffie et al., 2021; Weagle et al., 2018), including concentrations of secondary inorganic aerosols, black carbon, organic aerosols, and dust. These maps were created using a combination of AOD data and in situ measurements of PM chemical composition to constrain and downscale coarse CTM outputs (via LUR techniques) to spatial scales aligning with population density distributions. The resulting maps offer the possibility to assess the contributions of different anthropogenic emission sectors to regional and global health burdens (McDuffie et al., 2021; J. Chen et al., 2021; Shi et al., 2023).
These recent developments are a fundamental first step in comprehending the differential health effects of PM components. However, models are still directly reliant on AOD and in situ measurements; thus, they cannot forecast future PM concentrations and compositions and are limited in terms of identifying the sources of the organic fraction. To address this limitation, the atmospheric-science community should develop hybrid models that incorporate land use data with CTM outputs, retaining the source specificity and forecasting capabilities of the CTMs while benefiting from the fine-resolution information provided by land use data. In these models, in situ measurements should be utilized for model training rather than used as model inputs. CTMs have the added benefit of being able to quantify the sources of different constituents, which is especially valuable for the organic fraction. Overall, developing hybrid models that leverage the complementary strengths of CTMs and land use information will be key for providing exposure data suitable for determining the adverse health effects of individual PM components.
In this section, we present the types of field observations that, in our opinion, are required to accurately capture spatial and temporal variations in PM components, enabling the validation of exposure models.
4.1 Established monitoring networks of detailed PM chemical composition
Monitoring networks play a vital role in providing essential data for understanding the spatial distribution and long-term trends of air pollution, identifying emission sources, constraining human exposure models, and evaluating the effectiveness of emission reduction measures. International monitoring programmes, such as the SPARTAN2, EMEP3, IMPROVE4, ACTRIS5 and ASCENT6 programmes, have been critical in establishing and maintaining the operation of these networks. Besides providing continuous detailed PM measurements for policymaking, these monitoring programmes offer access to outstanding facilities and openly available databases for scientists from academia and the private sector, promoting cutting-edge science and international collaborations.
Another advantage of these programmes is the standardization of analytical approaches and data formats, which ensures data quality and comparability and facilitates data sharing and use. Data generated from these programmes may include particle number size distributions and the concentrations of elemental and organic carbon, major ions, and metal components. Figure 3 illustrates the distribution of stations across Europe from which we have gathered detailed information on PM chemical properties from various national and pan-European programmes. For some PM constituents, more than 100 000 daily concentrations at more than 500 sites are available, which is rare (if not unique). This is only possible thanks to the corresponding research infrastructures. Datasets of at least this scale are required to form a complete picture of the PM chemical and physical properties and sources, enabling our atmospheric-modelling community to optimize exposure maps and thus understand the differential health effects of PM constituents.
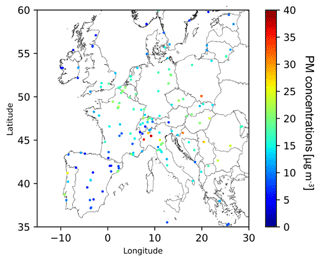
Figure 3European map showing site locations where long-term, detailed chemical-composition data are available. Sites include both urban and rural locations. Circles are colour-coded according to the total annual PM concentrations in 2013 to reflect the differences in emissions between sites.
The composition, emission sources, and formation pathways of the organic fraction remain a scientific challenge. Routine measurements (e.g. of organic carbon) are not sufficiently chemically resolved for retrieving the contributing sources. Instead, two approaches are currently used for long-term monitoring: the Aerosol Chemical Speciation Monitor (ACSM; Ng et al., 2011; Fröhlich et al., 2013), which measures the bulk composition of the non-refractory fraction of fine PM, and infrared spectroscopy (IR; Weakley et al., 2016), which measures the functional group composition of the organic fraction. We have utilized ACSM data to determine the contribution of residential emissions, vehicular emissions, and secondary processes to the organic aerosol fraction across Europe (Chen et al., 2022) and to validate CTM outputs (Ciarelli et al., 2017; Jiang et al., 2019). ACSM measurements are part of the ACTRIS and ASCENT programmes, whereas the IMPROVE network has adopted IR measurements. As the composition of the organic aerosols, especially that of the oxygenated secondary fraction, is complex, no single technique is complete. The spectra acquired with both ACSM and IR techniques retain information on the source origins and formation pathways of the organic fraction. These two techniques are complementary, and their combination, although currently only exploited in the laboratory (Yazdani et al., 2021, 2022), can be very powerful for further characterizing the organic aerosol fraction in dense networks over the long term, enabling a better understanding of the relationship between its composition and health effects.
Overall, it is essential that the scientific community continues to leverage chemically speciated PM data from monitoring networks and generates additional datasets for validating exposure models required to understand PM differential toxicity. These networks should include both urban and rural locations; this is key for accurately characterizing PM concentrations and chemical composition in different types of areas where people reside. It is also vitally important that governments continue investing in monitoring programmes to ensure the continuous acquisition of detailed air quality data.
4.2 Why detailed atmospheric chemistry matters: a comparison of severe PM pollution in northern China and northern India
Modest improvements in PM pollution in relatively clean regions in Western Europe and North America, where most of the current monitoring programmes operate, would result in significant reductions in mortality due to non-linear concentration–response relationships (Apte et al., 2015). In contrast, major improvements in air quality are required to substantially reduce mortality in more polluted regions, such as China and India (Fig. 4). However, such improvements are at least possible as high concentrations result from more controllable anthropogenic emissions. In China and India, air pollution is linked to 5 million deaths every year (Lelieveld et al., 2015), with approximately 20 % of these deaths attributable to PM (Fig. 5a). Projected demographic shifts in these regions indicate that in order to maintain current PM-attributable mortality rates, average PM levels must decrease by approximately 30 % within the next 15 years to counterbalance the rise in PM-related deaths resulting from ageing populations (Apte et al., 2015). Therefore, an effective programme for delivering clean air to polluted regions is urgently needed to prevent several million deaths every year.
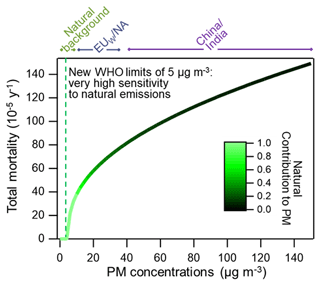
Figure 4Dose–response relationship between PM concentrations and total attributable mortality, highlighting the sensitivity of mortality to reductions in anthropogenic emissions at low and high pollution levels and, potentially, to contributions from natural emissions. The reproduction is adapted from Apte et al. (2015). The vertical axis indicates per capita mortality rates attributable to PM2.5 for a hypothetical global population that is uniformly exposed to a given level of PM2.5. The dose–response relationship is colour-coded according to the contribution of natural emissions to PM mass. For illustration, we have chosen a natural background concentration of 5 µg m−3, representing the level at which 50 % of the global population would be exposed if all anthropogenic emissions were eliminated (Pai et al., 2022). The horizontal bars at the top of the figure represent typical PM concentrations in Western Europe and/or North America (EUW/NA) and China and/or India, as well as natural background PM concentrations.
In response, China and India launched their country-level clean-air plans in 2013 and 2019, respectively. Despite greatly improved national air quality levels compared to 10 years ago (Fig. 5b), China is now finding it challenging to achieve further reductions in air pollution due to the trade-off between controlling PM and ozone pollution (Li et al., 2019). The situation in India is more alarming, with the country's air quality continuing to worsen despite the implementation of its clean-air programme. A growing number of cities are experiencing severe pollution (Ghildiyal, 2022), resulting in a rise in mortality attributable to PM (Fig. 5b). The mechanisms of haze formation in the two regions are also very different. While pollution in China occurs on regional scales, local pollution plays a prevailing role in India. The comparison between severe PM pollution in northern China and northern India serves as a perfect example of why a detailed understanding of the complex atmospheric chemistry involved is required to comprehend PM differential toxicity in those regions.
In China, secondary-aerosol production was identified as the main cause of winter haze events in a study conducted by Huang et al. (2014), which was the first of its kind to make such a discovery, doing so a decade ago. Subsequent studies have confirmed that, in Chinese megacities, particle formation, often observed at the onset of haze, is driven by the photochemical production of secondary organic and inorganic species, which occurs on a regional scale during the day (Yao et al., 2018; Kulmala et al., 2021). Multi-phase chemistry plays another essential role in aerosol formation during haze in China. The high concentrations of anthropogenic sulfate and nitrate, coupled with high relative humidity, provide a reactive medium for heterogeneous aerosol production (Tong et al., 2021). Under haze conditions, gaseous NO2 may also act as an oxidant in concentrated aqueous-aerosol media, producing additional secondary PM (Su et al., 2020; Wang et al., 2020). Compared with gas-phase photochemical reactions, which are self-buffered against heavy pollution, multi-phase chemistry exhibits positive feedback, which means that higher PM levels accelerate multi-phase production, further increasing PM concentrations (Le et al., 2020). Furthermore, due to the non-linear chemistry of ozone production and titration in winter, recent reductions in nitrogen oxides have resulted in ozone enhancement in urban areas (Li et al., 2019), further increasing the capacity of atmospheric oxidation and facilitating secondary-aerosol formation (Le et al., 2020). Substantial oxidation in China's atmosphere occurs even during the night. New findings reveal that between 2014 and 2019, decreases in pollution have led to an increase in the production rates of nitrate radicals across China, suggesting the growing role of nighttime chemistry in China's air pollution (H. Wang et al., 2023). Further mitigating air pollution and its health effects in China will require a detailed mechanistic understanding of the complex multi-phase and oxidation chemistries involved as well as the identification of the major sources of secondary-aerosol precursors.
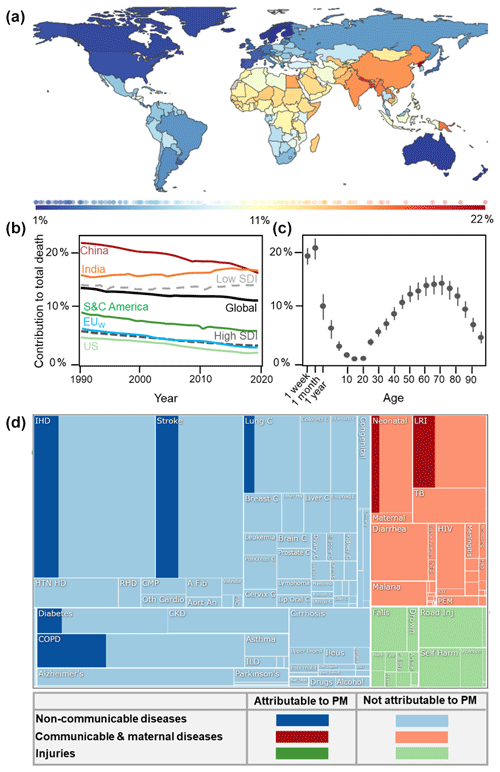
Figure 5Percentage of mortality attributable to particulate pollution. Data are from the results of the 2019 Global Burden of Disease study (Seattle, United States; Institute for Health Metrics and Evaluation, 2020) and are available at https://vizhub.healthdata.org/gbd-results/ (last access: 6 June 2023). (a) Percentage of PM-related mortality for each country. (b) Evolution of the percentage of PM-related mortality from 1990 to 2019 for locations discussed in the text, including China, India, Western Europe (EUW), the US, and South and Central America (S&C America); low SDI (socio-demographic index) levels; and high SDI levels. (c) Percentage of global PM-related mortality as a function of age. (d) Percentage of deaths attributable to PM pollution related to non-communicable diseases, communicable and maternal diseases, and injuries. The main causes of death to which PM exposure contributes include ischemic heart disease (IHD), stroke, diabetes, chronic obstructive pulmonary disease (COPD), neonatal infections, and lower respiratory infections (LRIs). HTN HD: hypertensive heart disease. RHD: rheumatic heart disease. CMP: cardiomyopathy. Oth Cardio: other cardiovascular diseases. CKD: chronic kidney disease. A Fib: atrial fibrillation. PAD: peripheral arterial disease. ILD: interstitial lung disease. C: cancer. IBD: inflammatory bowel disease. Vasc Intest: vascular intestinal disorders. Oth Digest: other digestive diseases. Hep: hepatitis. TB: tuberculosis. Typh + Paratyph: typhoid fever and paratyphoid fever. STI: sexually transmitted infection. Road inj: road injuries. Aort An: aortic aneurysm. Mech: mechanical incident. Other MN: other malignant responses. F Body: foreign body. Bac Skin: bacterial skin disease. PEM: protein energy malnutrition.
However, in Delhi, India, the rapid growth of particles to sizes relevant for haze formation occurs at night when no photochemistry is present. We have recently shown that the growth of sub-100 nm particles is predominantly driven by primary supersaturated organic vapours from local biomass combustion emissions, whose condensation is facilitated by the rapid decrease in air temperature and the increase in emissions during nighttime (Mishra et al., 2023). The formation of ammonium chloride enhances aerosol water uptake through co-condensation in the presence of high nighttime humidity, sustaining particle growth at greater sizes (Mishra et al., 2023) and leading to fog formation and a 50 % reduction in visibility (Gunthe et al., 2021). This process, apparently unique to India's capital, does not involve photochemistry but is instead driven by high emissions of hydrochloric acid, possibly from local industries (Rai et al., 2020). During daylight hours, as NOX emissions disperse and atmospheric oxidation capacity increases, local combustion of fossil fuels and biomass becomes an important source of SOA production (Kumar et al., 2022). Pollution levels in Delhi attributed toxic heavy metals are another cause for alarm, with concentrations reaching levels that are several hundred times higher than those found in Europe, also largely due to local industries (Rai et al., 2021). Addressing air pollution in India will require international collaboration with local researchers to better understand the local sources of different pollutants, e.g. through mobile measurements (Sect. 4.3), as well as the effects of local meteorological conditions on air quality. Given the significance of local pollution sources, it will also necessitate the involvement of social scientists and local communities to drive social changes and raise public awareness.
The atmospheric-science community has already made significant strides in understanding the sources of air pollution in China and India, but knowledge gaps still exist. It is imperative to further understand the non-linear effects of emissions on atmospheric oxidation capacity, particularly as India may face the same problems as China in the near future, when primary-pollution reduction could lead to an increase in the photochemical production of ozone and secondary aerosols. It is also crucial to identify, at a molecular level, the specific components contributing to aerosol formation and growth and to relate these components to the emission sources of their precursors. We also need to gain a mechanistic understanding of the interplay between the soluble inorganic fraction and water and the effects of both on the enhanced partitioning and heterogeneous chemistry of organic and inorganic vapours (e.g. N2O5, HCl, HNO3, and oxidized organics). Without this knowledge, we cannot accurately predict the fate of these vapours in light of future reductions in the anthropogenic emissions of inorganic precursors (SO2 and NOX). Detailed knowledge of atmospheric chemistry is key for understanding PM chemical composition and differential toxicity in these polluted regions.
Finally, it is essential to establish national monitoring networks in both countries that help us probe the spatial distribution and long-term trends of air pollution and allow us to evaluate the effectiveness of emission reduction measures. The data resulting from these monitoring programmes serve as a cornerstone for understanding the health effects of the PM components specific to China and India, enabling us to devise regionally specific solutions aimed at effectively limiting air pollution in these regions. More generally, the inequity of air pollution is flagrant as locations with a low socio-demographic index (SDI) suffer 3 times the burden of PM-related mortality compared to locations with a high SDI (Fig. 5b). This disparity underscores the urgent need for comprehensive monitoring programmes in low-SDI countries.
4.3 Urban mapping of PM chemical composition
Monitoring networks have limited spatial coverage, which can make it difficult to capture localized pollution hotspots, especially those arising from primary combustion emissions (Eeftens et al., 2012b; Elser et al., 2016, 2018; Jedynska et al., 2015, 2014). Therefore, several approaches have been proposed to enhance the spatial coverage of urban-pollution measurements (Fig. 2). Both ground-based sensor networks, e.g. those for CO2, black carbon, NO2, or total PM (Popoola et al., 2018; Caubel et al., 2019; Oney et al., 2015), and satellite retrievals (Di et al., 2016; Griffin et al., 2019) can map the concentrations of individual pollutants at sub-kilometre-scale resolutions; however, these approaches lack the chemical resolution needed for measurements of PM composition. Aircraft measurements are suited for studying pollution plumes at regional scales (Fry et al., 2018; Decker et al., 2019), but they cannot capture fine-scale variations at the ground level. Ground-based mobile laboratories can house online instrumentation that provides a high chemical resolution while operating with a sufficiently high time resolution (i.e. a few minutes) for measurements at street levels (Shairsingh et al., 2018; Gu et al., 2018). This makes them ideally suited for spatial mapping of PM composition in urban environments (Hankey and Marshall, 2015; Alexeeff et al., 2018; Apte et al., 2017; Gu et al., 2018).
A large number of studies have measured black carbon, NO2, total PM mass, and number concentrations aboard mobile platforms (Alexeeff et al., 2018; Apte et al., 2017; Hankey and Marshall, 2015; Shairsingh et al., 2018; Simon et al., 2017; Miller et al., 2020). The Aerodyne aerosol mass spectrometer (AMS) has also been used for the mobile measurements of non-refractory PM components, including secondary inorganic species and organic aerosols (Elser et al., 2016, 2018; Gu et al., 2018; Mohr et al., 2011; Shah et al., 2018). The application of factorization techniques to the measured organic mass spectra has enabled its apportionment to primary traffic, cooking, and biomass-burning emissions, as well as the quantification of the total secondary fraction (Gu et al., 2018; Elser et al., 2016, 2018). From measurements in the EU and the US, it has been found that secondary organic and inorganic fractions are homogeneously distributed across cities, while primary emissions are enhanced by several µg m−3 compared to background levels (Elser et al., 2016, 2018), aligning with land use variables (Gu et al., 2018).
Until recently, there has been no robust technology for highly time-resolved measurements of airborne particulate metals. Therefore, studies previously relied on integrated offline samples collected over days to weeks at a few sampling stations in order to assess the spatial distribution of yearly particulate metals across cities (H. Z. Li et al., 2016; Van Ryswyk et al., 2017; Zhang et al., 2015) and countries (J. Chen et al., 2020). With such measurements, intra-urban variability in metal concentrations can still be discerned. Recently, the Xact 625 Ambient Metals Monitor, an online X-ray fluorescence (XRF) spectrometer, has been developed and successfully deployed in the field for real-time measurements of particulate elements (∼25) with time resolutions as low as 30 min (Furger et al., 2017). Due to its high temporal resolution, sensitivity, and robustness in the field, the Xact spectrometer is capable of delivering several-month-long datasets of thousands of data points – 10–100 times more than offline techniques (Manousakas et al., 2022) – allowing for the retrieval of daily concentration patterns. However, further developments are needed to achieve particulate elemental analyses on timescales of minutes, suitable for mobile measurements. The availability of such measurements will be transformative, enabling access to PM elemental compositions at unparalleled resolutions and the development of more robust exposure models for metal components.
Street-level PM composition data can enhance, challenge, or confirm various air quality datasets used to retrieve PM differential toxicity, such as CTM outputs, land use regression predictions, and remotely sensed observations. This refinement can also aid in addressing the effect of human mobility in epidemiological studies (Zeger et al., 2000).
Human activities have profoundly altered the Earth's environment, impacting emissions, atmospheric composition, global temperatures, and land cover. In Fig. 6, we categorize the complex anthropogenic effects on PM composition into four broad classes:
-
Direct emissions make up the first class. These encompass both PM and anthropogenic PM precursors that are directly released into the atmosphere.
-
Land use changes form the second class and include changes in urban infrastructure, green initiatives, deforestation and/or forest management, and agricultural practices, all of which affect emissions and their accumulation patterns.
-
The third class involves the direct effects of anthropogenic emissions on the chemistry of natural PM. Here, pollutants from human activities react with biogenic emissions, leading to PM formation.
-
Indirect perturbation of natural PM makes up the fourth class. It is caused by anthropogenic emissions that impact natural ecosystems, inducing global warming, increased CO2 concentrations, shifts in vegetation patterns, and desertification.
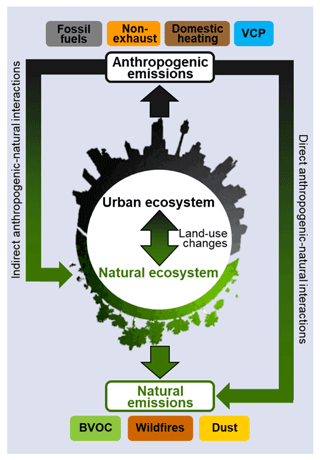
Figure 6Anthropogenic effects on PM through (1) direct emissions, (2) land use changes, (3) direct perturbation of natural PM, and (4) indirect perturbation of natural PM. The latter two comprise the direct effects of anthropogenic emissions on the chemistry of natural aerosols and the influence of anthropogenic emissions on natural ecosystems (e.g. through global warming or increases in CO2 concentrations), respectively. Natural emissions from terrestrial systems include biogenic volatile organic compounds (BVOCs), wildfire emissions, and dust. Anthropogenic emissions include NOX and SO2 from fossil fuel combustion, non-exhaust emissions, solid-fuel combustion for domestic heating, and volatile chemical products (VCPs).
This section addresses existing gaps in understanding anthropogenic emissions, their atmospheric transformation, and their direct and indirect influence on natural PM. It is crucial for the atmospheric-science community to approach these gaps from a mechanistic standpoint and incorporate them into models to accurately quantify the anthropogenic impacts on PM composition and, consequently, on health effects. In Sect. 5.1, we discuss anthropogenic PM sources that hold relevance for public health, while in Sect. 5.2, we examine the future trajectory of the natural PM background and its interactions with anthropogenic activities.
5.1 Legacy and emerging anthropogenic PM emissions
Anthropogenic emissions remain a predominant source of primary and secondary PM, posing a critical scientific and policy challenge in identifying the most harmful components to human health. Existing reviews have compiled epidemiological and toxicological evidence linking specific emissions to health endpoints (Wyzga and Rohr, 2015; Adams et al., 2015; Rohr and Wyzga, 2012; Yang et al., 2019; Morton Lippmann Lung, 2023). While there is extensive literature on short-term effects, particularly studies focusing on measurements at a few stations, longitudinal epidemiological studies investigating the effect of PM chemical composition on the outcomes of chronic health problems are relatively scarce. Despite inconsistencies across studies, elemental carbon, organic aerosols, sulfate, and metals have been consistently associated with increasing risks of cardiovascular and respiratory mortality and hospitalization (C. Chen et al., 2018; Yang et al., 2019; Masselot et al., 2022; Wang et al., 2022, 2017; Wyzga and Rohr, 2015; Adams et al., 2015; Rohr and Wyzga, 2012; Badaloni et al., 2017).
We believe that the principal challenge in establishing robust epidemiological associations with specific PM components lies in their correlation with other pollutants, such as other PM components (e.g. O3 and NOX). Therefore, we call for improved high-resolution large-scale chemically detailed exposure models that offer the necessary variability for overcoming limitations related to correlations. Moreover, we advocate for the continual development of epidemiological multi-component methods that estimate the joint health impacts of PM components rather than isolating the effects of individual impacts. In this section, we will focus on major anthropogenic emissions, including fossil fuel emissions, on-road non-exhaust emissions, volatile chemical products (VCPs), and residential biomass burning (Table 2).
Fossil fuel combustion is an important source of sulfate, nitrate, and elemental carbon. Numerous accounts have reported the higher differential toxicity of primary elemental-carbon emissions, especially that causing cardiovascular morbidity and mortality (H. Chen et al., 2020). Consequently, in 2021, the WHO listed elemental carbon (EC), or black carbon (BC), as one of the pollutants of emerging concern, calling for more evidence on their chronic health effects that can be used for future guidelines and regulations. To address this, it is vital to provide high-resolution national and continental BC maps suited for large-scale epidemiological studies (Sect. 3), ideally distinguishing between BC emissions caused by biomass burning and those caused by fossil fuel burning (Table 2).
Sulfate and nitrate are not toxic by themselves, and their high fraction in PM and extended spatial variation complicate the determination of their health effects. However, the toxicity of these secondary components is perhaps indirect, involving a complex multi-phase interplay with other components. Sulfate, originating from energy production emissions of SO2, provides an acidic medium for organic reactions and may increase the solubility (and hence the bioavailability) of metal particles, potentially increasing their toxicity. Mobile emissions of NOX have profound effects on atmospheric oxidation (Sect. 5.2) and also lead to enhanced partitioning (Lv et al., 2023) and subsequent multi-phase reactions of soluble organic molecules through nitrate formation. Traditionally, nitrate has been considered the chemical endpoint of the reactive-nitrogen life cycle in the atmosphere prior to wet or dry deposition. However, in recent years, there has been growing evidence of the photochemical renoxification of particulate nitrate in the presence of light and organic molecules (Jiang et al., 2023; Bao et al., 2020). While this process has mainly been examined for its potential to produce oxidant precursors (NOX and HONO), its effects on the composition of the organic fraction are currently not understood. A mechanistic understanding of these multi-phase processes, involving the interactions of secondary inorganic particles with organic and metal components, is indispensable for constraining their impact on PM chemical composition and differential toxicity (Table 2). There is a need for fundamental mechanistic investigations of these processes in the laboratory and in the field, especially in polluted areas, such as China, where multi-phase chemistry plays a key role in haze formation (Sect. 4.2).
As traffic exhaust emissions of NOX, PM, and hydrocarbons have become increasingly regulated, car engines have undergone a technological revolution, improving combustion efficiency and after-treatment technologies. In contrast, non-exhaust emissions, such as brake and tire wear, have increased with the growing number of vehicles and currently exceed exhaust emissions (Timmers and Achten, 2016). These emissions contain toxic metals, such as copper, which enhance PM oxidative potential (Daellenbach et al., 2020). Even with the electrification of vehicles, non-exhaust emissions will remain an issue and may worsen as a result of the heavier weight of electric cars (Timmers and Achten, 2016). While public transportation, including trams and trains, may also be an important source of metal particles, its contribution is not yet well quantified. Multi-site long-term measurements (Sect. 4.1) and mobile measurements that map trace element concentrations (Sect. 4.3) may offer new opportunities for representing the distribution of on-road non-exhaust emissions in exposure models (Table 2).
Table 2Future changes in anthropogenic emissions, key observations needed for coupling with health data, and high-priority model developments for understanding the health effects of anthropogenic emissions and their future evolution.
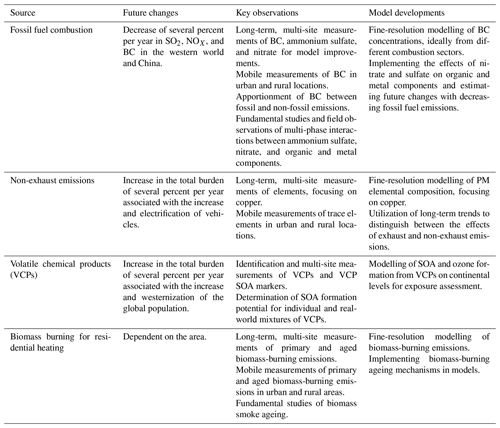
With the drastic reduction in emissions from on-road transportation, VCPs – which partly originate from indoor emissions – have emerged as one of the largest sources of outdoor urban organic emissions in US and European cities, modulating urban chemistry (Coggon et al., 2021; Gkatzelis et al., 2021; McDonald et al., 2018). These ubiquitous emissions stem from pesticides, coatings, printing inks, adhesives, cleaning agents, and personal care products. Human exposure to fossil carbonaceous aerosols may be shifting from transportation-related sources to VCPs. These emissions have comparable, if not greater, SOA potentials compared to vehicular emissions, which may influence human health. Variations in SOA potentials and chemistry among VCPs, as revealed by laboratory experiments, highlight the need for further characterization of these unconventional emissions (Shah et al., 2020). Furthermore, it is now possible to include these emissions in exposure models (Pennington et al., 2021). Existing regulations on VCPs emphasize reducing ozone and air toxics; however, numerous chemicals that contribute to SOA formation are currently exempt. Efforts to refocus mitigation strategies require atmospheric scientists to provide data quantifying the contribution of VCP emissions to SOAs and the global burden of disease (Table 2).
Achieving net-zero emissions for climate goals does not necessarily guarantee clean emissions for air quality. Biomass combustion, adopted as a carbon-neutral energy source for residential heating, is a potent anthropogenic source of pollution during winter. The emitted organic vapours rapidly react with OH and NO3 radicals in the atmosphere, resulting in substantial SOA production (Kodros et al., 2020; Stefenelli et al., 2019). The SOAs formed contain high levels of oxygenated and nitro-aromatic compounds, which likely cause the high oxidative potential of this fraction (Daellenbach et al., 2020). Recent laboratory investigations (Liu-Kang et al., 2022; S. Wang et al., 2023) and airborne field measurements (Morgan et al., 2020; Zhou et al., 2017) suggest that primary biomass emissions, which absorb near-UV light, can undergo photoreactions in the particle phase, resulting in a doubling of the emissions' oxidation state within a few hours. The dominant transformation processes of biomass-burning emissions and their impact on aerosol toxicity remain unclear. Overall, biomass smoke has not shown a reduction trend in many regions worldwide, underscoring the importance of comprehending the fate of these emissions in the atmosphere and their implications for human health (Table 2).
5.2 Anthropogenic effects on natural PM
With increasing regulations on anthropogenic emissions, the contribution of natural emissions – such as biogenic volatile organic compounds (BVOCs), wildfires, and desert dust – will gain prominence (Fig. 4). While these emissions stem from natural ecosystems, they are also significantly perturbed by anthropogenic activities, as illustrated in Fig. 6. The traditional picture that distinguishes between biogenic and anthropogenic sources obscures human impacts on ostensibly natural systems. Anthropogenic effects on natural PM can be either direct, through alterations to atmospheric reactivity, or indirect, through feedback mechanisms triggered by changes in the biosphere. We need to understand these effects quantitatively to devise best practices for mitigating their impacts. For example, the WHO's good practice statement on particles originating from sand and dust storms (referred to as “SDS” in the 2021 WHO air quality guidelines) includes measures that can be implemented to mitigate exposure. In this section, we discuss human influences on natural PM concentrations, chemical composition, and future trends (Table 3).
Biogenic SOAs (BSOAs) are the most important source of organic aerosols (OAs) in the atmosphere (Jiang et al., 2019), with anthropogenic NOX playing a vital role in moderating their formation and composition. NOX effects on BSOAs are multifaceted and involve (1) altering the fate of biogenic RO2 radicals, (2) increasing the atmospheric oxidant concentrations, and (3) providing an aqueous medium for additional multi-phase reactions (Xu et al., 2015; Pye et al., 2019; Carlton et al., 2018). As NOX emissions decrease, RO2 autoxidation becomes increasingly important, potentially enhancing BSOA formation, while the availability of oxidants driving RO2 formation rates simultaneously declines, possibly slowing regional BSOA formation. Recent modelling analyses (Carlton et al., 2018), along with in situ (Xu et al., 2015) and airborne measurements (Pye et al., 2019; Shrivastava et al., 2019) consistently suggest that anthropogenic NOX leads to a net enhancement of BSOA concentrations by 20 %–50 %, depending on the location and season. Similar to NOX, SO2 emissions from electricity generation, the main source of particulate sulfate, modulate the aqueous formation of isoprene SOAs. Models (Carlton et al., 2018) and measurements (Xu et al., 2015) across the US demonstrate that between 40 %–70 % of BSOAs can be controlled by reducing anthropogenic NOX and SO2. However, similar analyses are still lacking at other locations worldwide.
Table 3Future changes (2050–2100) in natural emissions, key observations needed for coupling with health data, and high-priority model developments for understanding the health effects of emissions and their future evolution.
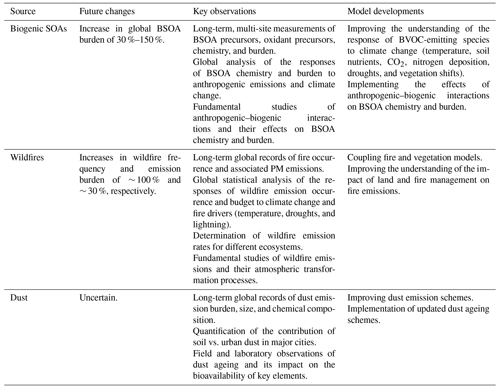
BSOA concentration exhibits a strong temperature dependence, driven by the exponential increase in BVOC emissions and their oxidation rates. Our analysis of multi-field observational datasets from European and North American locations reveals that BSOAs contribute (0.9–2.5) µg m−3 at 15 °C, compared to (2.1–6.3) µg m−3 at 25 °C (Xu et al., 2015; Daellenbach et al., 2017). Climate models project a global increase in BSOA mass by approximately 30 %–150 % with a temperature rise of 2 °C, along with an increase in atmospheric CO2 concentrations of a few hundred ppb (Carslaw et al., 2010). When changes in vegetation are accounted for, predictions of BVOC emissions become extremely uncertain, with projected increases ranging from tens to hundreds of percent (Table 3). These uncertainties arise from the unpredictable response of vegetation to future climates, including longer growing seasons, an increased leaf area index due to the fertilization effect of CO2, changes in water stress, and the expansion of boreal and temperate forests. With the rise in BVOC emissions and the denitrification of the atmosphere, it is expected that the oxidation capacity of the atmosphere may decrease, leading to slower production of BSOAs and a complete change in their composition. Understanding the non-linear interactions among anthropogenic emissions of oxidant precursors, greenhouse gases, atmospheric oxidation conditions, and the biosphere is crucial for understanding the concentration, chemical composition, and health effects of BSOAs, as well as future concentration trends in different regions.
While reducing NOX and SO2 can control a significant portion of BSOAs, the rise in BVOC emissions due to climate change, though highly uncertain, may offset this reduction. Currently, there is very limited understanding of the impact of BSOAs on human health, with only one study suggesting a 3.5-fold increase in cardiorespiratory mortality associated with anthropogenically influenced BSOAs compared to PM2.5 mass (Pye et al., 2021). Atmospheric scientists should capitalize on emerging multi-year, multi-location observations of detailed PM chemistry to enhance model predictions of BSOAs (Table 3).
Wildfires have become increasingly frequent in many regions worldwide, making them the second-largest contributor to atmospheric organic carbon on a global scale. This source can be affected directly by anthropogenic activities, such as deforestation, forest management, and fire suppression, or indirectly by climate change. Climate models predict that global warming will amplify wildfire emissions by ∼ 30 % as a result of longer fire seasons, higher temperatures, increased droughts, and increased usage of convection-induced lightning as an ignition source (Carslaw et al., 2010). Studies have discussed the short-term health effects of PM from wildfire emissions, including pulmonary complications (Stawovy and Balakrishnan, 2022), respiratory mortality and cardiovascular mortality (G. Chen et al., 2021), whereas understanding the long-term health effects of these emissions remains an ongoing area of research (Grant and Runkle, 2022; Gao et al., 2023). Recent studies investigating Amazonian (Yu et al., 2022) and Canadian Boreal (Korsiak et al., 2022) wildfire emissions have highlighted an elevated risk of various cancers, surpassing the effects of non-wildfire PM emissions at equivalent exposure doses. A crucial first step in improving predictions of wildfire emissions and their health effects involves analysing global fire occurrence records and associated PM emissions, establishing robust relationships between emissions, ecosystems, climate change, fire management, and fire drivers (Table 3).
Another naturally occurring PM component, dust, is the most important source of elements in the atmosphere. Wind speed, soil moisture, and vegetation cover are the main drivers of dust emission fluxes, size distribution, and mineralogical composition (Carslaw et al., 2010). During transport, dust particles react with acids, increasing the bioavailability of key elements, including iron. It has been shown that anthropogenic sulfate from fossil fuel combustion modulates soil dust iron solubility and toxicity (Wong et al., 2020). The extent to which gases react with dust particles heavily depends on the mineralogical composition of the dust, with particles rich in carbonates exhibiting strong atmospheric reactivity. Like wildfires, both direct human activities and climate change can influence dust emissions, making future predictions uncertain (Carslaw et al., 2010). Dust outbreaks have frequently been associated with mortality and hospital admissions (Stafoggia et al., 2016; Crooks et al., 2016), albeit with moderate effects and high uncertainties (Zhang et al., 2016). This uncertainty may result from the large variability in dust particle morphology, size, solubility, and chemical composition, depending on the particles' origin and transport time in the atmosphere. A particular challenge is the provision of long-term, large-scale datasets, which is crucial because of the strong spatial and temporal variability in dust particles in the atmosphere. Therefore, additional observational data on dust phenomenology are required for model evaluation, which will help us understand the toxicity of dust and its contributing factors. Finally, fundamental research on dust transformation processes is also warranted (Table 3).
While anthropogenic emissions are expected to decrease, natural emissions will most likely increase. Part of this increase can be controlled by reducing anthropogenic emissions and managing land use. The atmospheric-science community is now ready to provide the field measurements, laboratory observations, and model outputs needed to quantify the global contributions of anthropogenic and controllable natural emissions and predict their evolution with global changes. This data will form the foundation for understanding the toxicity of anthropogenic PM sources and determining the natural PM background in different regions.
As aerosol scientists, we must shift our focus from seeking a single, elusive chemical or toxicological metric that describes PM health effects to providing chemically resolved atmospheric observations and modelling outputs that can establish epidemiological links between PM components and diseases. Epidemiology drives regulatory changes, and by working with epidemiologists, atmospheric scientists can assume a central role in determining PM composition and spatial distribution to unlock a better understanding of the complex epidemiological relationships between PM composition and health outcomes. This section highlights some notable advancements in modern environmental epidemiology that may not yet be fully appreciated within the atmospheric-chemistry community.
6.1 Collaboration between atmospheric scientists and epidemiologists
Recently, eight hallmarks of environmental insults have been proposed (Peters et al., 2021). They encompass oxidative stress and inflammation, genomic mutations, epigenetic alterations, mitochondrial dysfunction, endocrine disruption, altered intercellular communication, changes in microbiome communities, and impaired nervous system function. These hallmarks jointly underpin the health effects resulting from lifelong environmental exposures, including exposures to relatively modest concentrations of contaminants.
PM, as one of the most important environmental insults, can infiltrate our bodies through various barriers, e.g. our lungs or digestive system, affecting individuals through a complex web of biological pathways. Figure 5c illustrates the contribution of PM pollution to total mortality across various ages, highlighting its staggering impact on infants and the elderly. Short-term exposure has been linked to sudden infant death syndrome as well as increased mortality and morbidity rates due to cardiorespiratory and renal complications, particularly affecting children and individuals with chronic conditions (Heft-Neal et al., 2018; Zhang et al., 2023; Liu et al., 2023; Guo et al., 2023). Meanwhile, long-term exposures have been linked to numerous non-communicable diseases that manifest at a later stage of life (Fig. 5c), including cardiovascular diseases (Requia et al., 2017; Lelieveld et al., 2019), respiratory symptoms, different types of cancers (Turner et al., 2020), diabetes (Yang et al., 2018), and neurodegenerative diseases (Maher et al., 2016; Shi et al., 2020).
Unlike infectious diseases, non-communicable diseases have multiple causes and involve various factors, which individually may neither be necessary nor sufficient to cause the disease. Early-life exposures may leave enduring marks on the body, leading to manifestations that emerge many decades later. Given the multifactorial nature of the problem, epidemiology is irreplaceable when it comes to investigating PM-related non-communicable diseases, and working with citizen cohorts is essential for circumventing the challenges of randomization and confounding effects. Citizen involvement is simply crucial for individuals to understand their own health.
To bolster the causal interpretation of epidemiological associations derived from observational studies, it is crucial to elucidate how the hallmarks of environmental insults connect exposure to disease development. This requires a robust methodological approach that combines insights from aerosol science into PM chemical composition, sources, and spatial distribution with modern environmental-epidemiology techniques, including molecular phenotyping, multiomics, epigenetics, imaging, and personalized and digital medicine.
6.2 Working with citizen cohorts to establish causal links
The establishment and maintenance of national biobanks and citizen cohorts are key for investigating the causal links between exposure to PM components and diseases. These cohorts represent the gold standard for understanding the long-term health effects of environmental factors (Probst-Hensch et al., 2022). They provide evidence in situations where randomized trials are unethical or unfeasible (Peters et al., 2022). Cohorts are critical for developing a causal understanding of how social, environmental, behavioural, and economic factors promote or hinder health and for evaluating the long-term impacts of public-health interventions. They enable the study of health trajectories across different ages, providing a life course perspective. As such, they serve as a fundamental pillar for addressing the health effects of PM in the context of other major public-health challenges of the 21st century, including population growth, ageing societies, urbanization, global warming, digital transformation, and increasing social inequalities.
As highlighted in Probst-Hensch et al. (2022), Europe has a long-standing tradition of implementing and maintaining large-scale (> 100 thousand participants) and long-term (> 20 years) cohorts, including the UK Biobank (Sudlow et al., 2015), Lifelines (Stolk et al., 2008), Constances (Zins et al., 2010), and the German National Cohort (Peters et al., 2022). Innovations in these cohorts include recruitment from birth to old age, the implementation of novel eHealth tools, the involvement of psychologists and social scientists, and citizen participation during planning and execution to address response rate challenges. Biomaterial collection within these cohorts enables sequencing and in-depth molecular characterization, differentiating between genetic and environmental factors.
A noteworthy addition to national cohorts is the global mortality dataset maintained by the Global Burden of Disease Collaborative Network, within the Institute for Health Metrics and Evaluation (see Fig. 5). Although the dataset is limited to cause-specific mortality, this network has shaped modern epidemiology and allowed for the quantification of the global burden of PM mortality (Burnett et al., 2018). Another emerging dataset is from the multi-country, multi-city network, which provides daily mortality data for several locations around the world, ideal for assessing short-term PM exposures (Masselot et al., 2022; Liu et al., 2019). Such datasets synergistically complement causal investigations into PM health effects based on cohort data, providing a global perspective.
Our vision involves closer collaboration that allows for detailed knowledge of PM composition and exposure to be integrated into citizen cohorts, with an increasing focus on polluted areas, such as China and India (Sect. 4.2). Working together will advance the understanding of how specific PM components or combinations of components contribute to disease development and facilitate the detection of early changes resulting from exposure. This collaboration will generate even more compelling evidence for science-to-citizen-to-policy partnerships, which are needed to influence regulatory frameworks.
6.3 Disease prevention through the mitigation of detrimental PM components
It is vital to prioritize quality of life and healthy ageing over simply extending life expectancy, especially in high-SDI regions. This necessitates a shift towards primary prevention and the implementation of drastic changes in health promotion, starting in childhood and early adulthood, well before the onset of diseases. In the case of PM, it is essential to identify and mitigate the specific components responsible for different diseases to alleviate their impacts on our well-being. A reduction in detrimental PM components will also result in an extension of life expectancy, especially in low-SDI locations.
Dementia serves as a prime example of the major challenges facing our ageing society. Dementia is a severe decline in cognitive function that considerably affects the well-being of older adults and their families and imposes substantial costs on public programmes. In 2010, approximately 115 million adults were living with dementia worldwide (Prince et al., 2013), resulting in an estimated economic impact of USD 600 billion (Wimo et al., 2013). Given the sharp rise in dementia beyond the age of 75 and our increasingly ageing society, global dementia cases are forecasted to triple by the year 2050. Recent studies in the US have shown that every 5 µg m−3 increase in annual PM concentrations results in a 13 % increase in the risk of first-time hospital admissions for dementia (Shi et al., 2020), with elemental-carbon and sulfate particles having the strongest effects (Shi et al., 2023). While more research, including studies at other locations, is necessary to confirm these associations and understand the underlying biological pathways involved, these studies already shed light on possible interventions for slowing the trajectory of cognitive decline and ensuring the well-being of our ageing society. This example reinforces that a collaborative effort that combines the expertise of epidemiologists in understanding disease patterns with the experience of atmospheric scientists in measuring and modelling PM composition is crucial for mitigating the impact of PM components on the well-being of our society. Such collaboration will also help identify the PM components contributing to other diseases, potentially revealing distinct biological pathways involved in their development.
In the 21st century, we have witnessed a remarkable rise in life expectancy and shifts in global disease patterns, attributable to a combination of public-health interventions and advancements in healthcare and healthcare accessibility. Yet, 10 million deaths attributable to environmental exposures can still be prevented every year (Neira and Prüss-Ustün, 2016; Landrigan et al., 2018), highlighting the need for proactive measures. Relying solely on high-tech medical interventions for managing disease progression may exacerbate existing social inequalities within healthcare systems and yield diminishing returns. Therefore, we advocate for a shift towards enhancing quality of life and promoting healthy ageing through early prevention and the creation of healthy environments for all. Our vision for realizing this goal involves close collaboration between atmospheric scientists and epidemiologists to integrate chemically detailed data on global air quality with large-scale sources of personalized medical information from citizen cohorts. The provision of global PM composition maps will require the atmospheric-science community to (1) develop spatially and chemically detailed exposure models, (2) provide long-term time series of PM chemical composition from monitoring networks, (3) map pollution hot-spots through mobile measurements, (4) understand emerging anthropogenic emissions and their chemical transformations, especially in heavily polluted areas (such as China and India), and (5) understand the future evolution of natural emissions with climate and land use changes.
As an aggressive attempt to promote healthy environments, the WHO has set new guidelines to limit PM concentrations to below 5 µg m−3. Achieving these limits may be challenging for many regions due to the contributions of natural emissions from wildfires, biogenic species, and desert dust. Concurrently, scientific consensus underscores the critical role of PM chemical composition in influencing associated health effects, necessitating a re-evaluation of how we should mitigate PM pollution and the development of new generation of air quality metrics focusing on detrimental PM components. Focusing on the differential toxicity of PM offers two key advantages. First, it allows for targeted measures aimed at limiting specific health-relevant PM sources. Second, PM chemical composition is intertwined with other properties that may also drive the health effects of PM, such as solubility, number size distribution, and oxidative potential. Atmospheric science has reached a pivotal moment that allows for the provision of detailed global air quality maps at a sufficiently fine resolution, supporting epidemiological studies in their aim to determine the differential toxicity of PM components. This is crucial for integrating PM chemical composition into regulatory frameworks, informing targeted policymaking and operational decisions.
Routine, widespread availability of high-resolution atmospheric data could have transformative implications for air quality research, epidemiology, and environmental management. These valuable data can reveal localized pollution hotspots, offering new opportunities for implementing targeted pollution control measures. When combined with personal GPS data, they enable the development of comprehensive personalized exposure analytics, potentially influencing individual behaviour. This parallels the way real-time traffic data currently shape driving patterns at an individual level or how health applications motivate individuals to engage in active exercise.
By providing open access to global high-resolution pollution maps, atmospheric scientists can assume a broader societal role in raising public awareness of air pollution and its impacts on health and environmental equity. These pollution maps equip citizens, local communities and policymakers with the tools needed to optimize emission reduction, strategies and sustainable urban planning. This can include the application of targeted measures for limiting the most important PM sources to protect our health or shifts in urban land use design for better air quality. This wealth of data can be utilized to train models that predict the future evolution of air pollution sources and their health impacts with regard to climate change, land use change, urban planning, mitigation strategies, and energy policies. Long-term global air quality data are a key cornerstone for establishing targeted strategies to improve public health and anticipate its future trajectory.
In the process of re-evaluating and implementing air quality guidelines, a multidisciplinary collaborative approach involving atmospheric scientists, climate scientists, toxicologists, exposure scientists, epidemiologists, public-health experts, social scientists, policymakers, and the public is crucial. Therefore, governments must ensure sustainable funding to foster these collaborations as the benefits in terms of lives saved and costs reduced are becoming increasingly evident. By alleviating the burden of air-pollution-related diseases, we can prioritize the health and well-being of individuals and create sustainable and resilient communities.
No code or software other than Microsoft Office has been used.
We are creating and continuously maintaining an aerosol chemistry database based on available and ongoing measurements. The observational data cover more than 500 sites, with more than 100 000 daily concentrations for some components from 2008 to the present. In addition, complementary outputs for chemical transport modelling are available for the same period within the European domain. This dataset is a work in progress and will eventually be made openly accessible to the community. Specific parts of the database can already be shared with atmospheric scientists, air quality and climate modellers, and epidemiologists upon request to the corresponding author.
IEH wrote the paper and produced the figures. All authors commented on the paper.
The contact author has declared that none of the authors has any competing interests.
Publisher’s note: Copernicus Publications remains neutral with regard to jurisdictional claims made in the text, published maps, institutional affiliations, or any other geographical representation in this paper. While Copernicus Publications makes every effort to include appropriate place names, the final responsibility lies with the authors.
This article is part of the special issue “20 years of Atmospheric Chemistry and Physics”. It is not associated with a conference.
We thank the Global Burden of Disease Collaborative Network and the Institute for Health Metrics and Evaluation (Seattle, United States) for providing the data used in Fig. 5 (available at https://vizhub.healthdata.org/gbd-results/, last access: 6 June 2023).
The Swiss Data Science Centre (AURORA; project no. C20-08), the Swiss Federal Office for the Environment (FOEN), and the Swiss National Science Foundation (SNSF; grants no. 189883 and PZPGP2_201992) provided financial support for this work. We also thank the Swiss Agency for Development and Cooperation (SDC) for financially supporting the Clean Air Project in India (grant no. 7F-10093.01.04) and the Clean Air China Programme (grant no. 7F-09802.01.03).
This paper was edited by James Allan and Barbara Ervens and reviewed by two anonymous referees.
Adams, K., Greenbaum, D. S., Shaikh, R., van Erp, A. M., and Russell, A. G.: Particulate matter components, sources, and health: Systematic approaches to testing effects, J. Air Waste Manage. Assoc., 65, 544–558, https://doi.org/10.1080/10962247.2014.1001884, 2015.
Alderete, T. L., Jones, R. B., Chen, Z., Kim, J. S., Habre, R., Lurmann, F., Gilliland, F. D., and Goran, M. I.: Exposure to traffic-related air pollution and the composition of the gut microbiota in overweight and obese adolescents, Environ. Res., 161, 472–478, https://doi.org/10.1016/j.envres.2017.11.046, 2018.
Alexeeff, S. E., Roy, A., Shan, J., Liu, X., Messier, K., Apte, J. S., Portier, C., Sidney, S., and Van Den Eeden, S. K.: High-resolution mapping of traffic related air pollution with Google street view cars and incidence of cardiovascular events within neighborhoods in Oakland, CA, Environ. Health, 17, 38, https://doi.org/10.1186/s12940-018-0382-1, 2018.
Apte, J. S., Marshall, J. D., Cohen, A. J., and Brauer, M.: Addressing Global Mortality from Ambient PM2.5, Environ. Sci. Technol., 49, 8057–8066, https://doi.org/10.1021/acs.est.5b01236, 2015.
Apte, J. S., Messier, K. P., Gani, S., Brauer, M., Kirchstetter, T. W., Lunden, M. M., Marshall, J. D., Portier, C. J., Vermeulen, R. C. H., and Hamburg, S. P.: High-Resolution Air Pollution Mapping with Google Street View Cars: Exploiting Big Data, Environ. Sci. Technol., 51, 6999–7008, https://doi.org/10.1021/acs.est.7b00891, 2017.
Badaloni, C., Cesaroni, G., Cerza, F., Davoli, M., Brunekreef, B., and Forastiere, F.: Effects of long-term exposure to particulate matter and metal components on mortality in the Rome longitudinal study, Environ. Int., 109, 146–154, https://doi.org/10.1016/j.envint.2017.09.005, 2017.
Bailey, M. J., Naik, N. N., Wild, L. E., Patterson, W. B., and Alderete, T. L.: Exposure to air pollutants and the gut microbiota: a potential link between exposure, obesity, and type 2 diabetes, Gut Microbes, 11, 1188–1202, https://doi.org/10.1080/19490976.2020.1749754, 2020.
Bao, F., Jiang, H., Zhang, Y., Li, M., Ye, C., Wang, W., Ge, M., Chen, C., and Zhao, J.: The Key Role of Sulfate in the Photochemical Renoxification on Real PM2.5, Environ. Sci. Technol., 54, 3121–3128, https://doi.org/10.1021/acs.est.9b06764, 2020.
Bayer-Oglesby, L., Schindler, C., Hazenkamp-von Arx, M. E., Braun-Fahrländer, C., Keidel, D., Rapp, R., Künzli, N., Braendli, O., Burdet, L., Sally Liu, L. J., Leuenberger, P., and Ackermann-Liebrich, U.: Living near main streets and respiratory symptoms in adults: the Swiss Cohort Study on Air Pollution and Lung Diseases in Adults, Am. J. Epidemiol., 164, 1190–1198, https://doi.org/10.1093/aje/kwj338, 2006.
Bell, M. L., Davis, D. L., and Fletcher, T.: A retrospective assessment of mortality from the London smog episode of 1952: the role of influenza and pollution, Environ. Health Perspect., 112, 6–8, https://doi.org/10.1289/ehp.6539, 2004.
Boogaard, H., Patton, A. P., Atkinson, R. W., Brook, J. R., Chang, H. H., Crouse, D. L., Fussell, J. C., Hoek, G., Hoffmann, B., Kappeler, R., Kutlar Joss, M., Ondras, M., Sagiv, S. K., Samoli, E., Shaikh, R., Smargiassi, A., Szpiro, A. A., Van Vliet, E. D. S., Vienneau, D., Weuve, J., Lurmann, F. W., and Forastiere, F.: Long-term exposure to traffic-related air pollution and selected health outcomes: A systematic review and meta-analysis, Environ. Int., 164, 107262, https://doi.org/10.1016/j.envint.2022.107262, 2022.
Brauer, M., Brook, J. R., Christidis, T., Chu, Y., Crouse, D. L., Erickson, A., Hystad, P., Li, C., Martin, R. V., Meng, J., Pappin, A. J., Pinault, L. L., Tjepkema, M., van Donkelaar, A., Weichenthal, S., and Burnett, R. T.: Mortality-air pollution associations in low-exposure environments (MAPLE): Phase 1, Health Effects Institute, Boston, MA, PMID: https://www.healtheffects.org/system/files/brauer-rr-203-phase1-report_2.pdf (last access: 23 August 2024), 1–87, 2019.
Brokamp, C., Jandarov, R., Rao, M. B., LeMasters, G., and Ryan, P.: Exposure assessment models for elemental components of particulate matter in an urban environment: A comparison of regression and random forest approaches, Atmos. Environ., 151, 1–11, https://doi.org/10.1016/j.atmosenv.2016.11.066, 2017.
Brown, J. R. and Thornton, J. L.: Percivall Pott (1714–1788) and Chimney Sweepers, Cancer of the Scrotum, Brit. J. Ind. Med., 14, 68–70, https://doi.org/10.1136/oem.14.1.68, 1957.
Brunekreef, B. and Holgate, S. T.: Air pollution and health, The Lancet, 360, 1233–1242, https://doi.org/10.1016/S0140-6736(02)11274-8, 2002.
Brunekreef, B. S., M. Chen, J. Andersen, Z. J., Atkinson, R., Bauwelinck, M., Bellander, T., Boutron, M. C., Brandt, J., Carey, I., Cesaroni, G., Forastiere, F., Fecht, D., Gulliver, J., Hertel, O., Hoffmann, B., de Hoogh, K., Houthuijs, D., Hvidfeldt, U., Janssen, N., Jørgensen, J., Katsouyanni, K., Ketzel, M., Klompmaker, J., Krog, N. H., Lui, S., Ljungman, P., Mehta, A., Nagel, G., Oftedal, B., Pershagen, G., Peters, A., Raaschou-Nielsen, O., Renzi, M., Rodopoulou, S., Samoli, E., Schwarze, P., Sigsgaard, T., Stafoggia, M., Vienneau, D., Weinmayr, G., Wolf, K. and Hoek, G.: Mortality and Morbidity Effects of Long-Term Exposure to Low-Level PM2.5, BC, NO2, and O3: An Analysis of European Cohorts in the ELAPSE Project. Res Rep Health Effect Institute 2021 September, 2021(208), 1–127, PMID: 36106702; PMCID: PMC9476567, 2021.
Burnett, R. T., Arden Pope III, C., Ezzati, M., Olives, C., Lim, S. S., Mehta, S., Shin, H. H., Singh, G., Hubbell, B., Brauer, M., Anderson, H. R., Smith, K. R., Balmes, J. R., Bruce, N. G., Kan, H., Laden, F., Prüss-Ustün, A., Turner, M. C., Gapstur, S. M., Ryan Diver, W., and Cohen, A.: An Integrated Risk Function for Estimating the Global Burden of Disease Attributable to Ambient Fine Particulate Matter Exposure, Environ. Health Perspect., 122, 397–403, https://doi.org/10.1289/ehp.1307049, 2014.
Burnett, R., Chen, H., Szyszkowicz, M., Fann, N., Hubbell, B., Pope, C. A., Apte, J. S., Brauer, M., Cohen, A., Weichenthal, S., Coggins, J., Di, Q., Brunekreef, B., Frostad, J., Lim, S. S., Kan, H., Walker, K. D., Thurston, G. D., Hayes, R. B., Lim, C. C., Turner, M. C., Jerrett, M., Krewski, D., Gapstur, S. M., Diver, W. R., Ostro, B., Goldberg, D., Crouse, D. L., Martin, R. V., Peters, P., Pinault, L., Tjepkema, M., van Donkelaar, A., Villeneuve, P. J., Miller, A. B., Yin, P., Zhou, M., Wang, L., Janssen, N. A. H., Marra, M., Atkinson, R. W., Tsang, H., Quoc Thach, T., Cannon, J. B., Allen, R. T., Hart, J. E., Laden, F., Cesaroni, G., Forastiere, F., Weinmayr, G., Jaensch, A., Nagel, G., Concin, H., and Spadaro, J. V.: Global estimates of mortality associated with long-term exposure to outdoor fine particulate matter, P. Natl. Acad. Sci. USA, 115, 9592, https://doi.org/10.1073/pnas.1803222115, 2018.
Carlton, A. G., Pye, H. O. T., Baker, K. R., and Hennigan, C. J.: Additional Benefits of Federal Air-Quality Rules: Model Estimates of Controllable Biogenic Secondary Organic Aerosol, Environ. Sci. Technol., 52, 9254–9265, https://doi.org/10.1021/acs.est.8b01869, 2018.
Carslaw, K. S., Boucher, O., Spracklen, D. V., Mann, G. W., Rae, J. G. L., Woodward, S., and Kulmala, M.: A review of natural aerosol interactions and feedbacks within the Earth system, Atmos. Chem. Phys., 10, 1701–1737, https://doi.org/10.5194/acp-10-1701-2010, 2010.
Cattani, G., Gaeta, A., Di Menno di Bucchianico, A., De Santis, A., Gaddi, R., Cusano, M., Ancona, C., Badaloni, C., Forastiere, F., Gariazzo, C., Sozzi, R., Inglessis, M., Silibello, C., Salvatori, E., Manes, F., and Cesaroni, G.: Development of land-use regression models for exposure assessment to ultrafine particles in Rome, Italy, Atmos. Environ., 156, 52–60, https://doi.org/10.1016/j.atmosenv.2017.02.028, 2017.
Caubel, J. J., Cados, T. E., Preble, C. V., and Kirchstetter, T. W.: A Distributed Network of 100 Black Carbon Sensors for 100 Days of Air Quality Monitoring in West Oakland, California, Environ. Sci. Technol., 53, 7564–7573, https://doi.org/10.1021/acs.est.9b00282, 2019.
Chen, C. and Zhao, B.: Review of relationship between indoor and outdoor particles: I/O ratio, infiltration factor and penetration factor, Atmos. Environ., 45, 275–288, https://doi.org/10.1016/j.atmosenv.2010.09.048, 2011.
Chen, C., Xu, D., He, M. Z., Wang, Y., Du, Z., Du, Y., Qian, Y., Ji, D., and Li, T.: Fine Particle Constituents and Mortality: A Time-Series Study in Beijing, China, Environ. Sci. Technol., 52, 11378–11386, https://doi.org/10.1021/acs.est.8b00424, 2018.
Chen, G., Canonaco, F., Tobler, A., Aas, W., Alastuey, A., Allan, J., Atabakhsh, S., Aurela, M., Baltensperger, U., Bougiatioti, A., De Brito, J. F., Ceburnis, D., Chazeau, B., Chebaicheb, H., Daellenbach, K. R., Ehn, M., El Haddad, I., Eleftheriadis, K., Favez, O., Flentje, H., Font, A., Fossum, K., Freney, E., Gini, M., Green, D. C., Heikkinen, L., Herrmann, H., Kalogridis, A.-C., Keernik, H., Lhotka, R., Lin, C., Lunder, C., Maasikmets, M., Manousakas, M. I., Marchand, N., Marin, C., Marmureanu, L., Mihalopoulos, N., Moènik, G., Nêcki, J., O'Dowd, C., Ovadnevaite, J., Peter, T., Petit, J.-E., Pikridas, M., Matthew Platt, S., Pokorná, P., Poulain, L., Priestman, M., Riffault, V., Rinaldi, M., Ró?`añski, K., Schwarz, J., Sciare, J., Simon, L., Skiba, A., Slowik, J. G., Sosedova, Y., Stavroulas, I., Styszko, K., Teinemaa, E., Timonen, H., Tremper, A., Vasilescu, J., Via, M., Vodièka, P., Wiedensohler, A., Zografou, O., Cruz Minguillón, M., and Prévôt, A. S. H.: European aerosol phenomenology – 8: Harmonised source apportionment of organic aerosol using 22 Year-long ACSM/AMS datasets, Environ. Int., 166, 107325, https://doi.org/10.1016/j.envint.2022.107325, 2022.
Chen, G., Guo, Y., Yue, X., Tong, S., Gasparrini, A., Bell, M. L., Armstrong, B., Schwartz, J., Jaakkola, J. J. K., Zanobetti, A., Lavigne, E., Nascimento Saldiva, P. H., Kan, H., Royé, D., Milojevic, A., Overcenco, A., Urban, A., Schneider, A., Entezari, A., Vicedo-Cabrera, A. M., Zeka, A., Tobias, A., Nunes, B., Alahmad, B., Forsberg, B., Pan, S.-C., Íñiguez, C., Ameling, C., De la Cruz Valencia, C., Åström, C., Houthuijs, D., Van Dung, D., Samoli, E., Mayvaneh, F., Sera, F., Carrasco-Escobar, G., Lei, Y., Orru, H., Kim, H., Holobaca, I.-H., Kyselý, J., Teixeira, J. P., Madureira, J., Katsouyanni, K., Hurtado-Díaz, M., Maasikmets, M., Ragettli, M. S., Hashizume, M., Stafoggia, M., Pascal, M., Scortichini, M., de Sousa Zanotti Stagliorio Coêlho, M., Valdés Ortega, N., Ryti, N. R. I., Scovronick, N., Matus, P., Goodman, P., Garland, R. M., Abrutzky, R., Garcia, S. O., Rao, S., Fratianni, S., Dang, T. N., Colistro, V., Huber, V., Lee, W., Seposo, X., Honda, Y., Guo, Y. L., Ye, T., Yu, W., Abramson, M. J., Samet, J. M., and Li, S.: Mortality risk attributable to wildfire-related PM2.5 pollution: a global time series study in 749 locations, The Lancet Planetary Health, 5, e579–e587, https://doi.org/10.1016/S2542-5196(21)00200-X, 2021.
Chen, H., Zhang, Z., van Donkelaar, A., Bai, L., Martin, R. V., Lavigne, E., Kwong, J. C., and Burnett, R. T.: Understanding the Joint Impacts of Fine Particulate Matter Concentration and Composition on the Incidence and Mortality of Cardiovascular Disease: A Component-Adjusted Approach, Environ. Sci. Technol., 54, 4388–4399, https://doi.org/10.1021/acs.est.9b06861, 2020.
Chen, J. and Hoek, G.: Long-term exposure to PM and all-cause and cause-specific mortality: A systematic review and meta-analysis, Environ. Int., 143, 105974, https://doi.org/10.1016/j.envint.2020.105974, 2020.
Chen, J., Hoogh, K. d., Strak, M., Kerckhoffs, J., Vermeulen, R., Brunekreef, B., and Hoek, G.: OP III – 4 Exposure assessment models for no2 and pm2.5 in the elapse study: a comparison of supervised linear regression and machine learning approaches, J. Occup. Environ. Med., 75, A6–A-6, https://doi.org/10.1136/oemed-2018-ISEEabstracts.14, 2018.
Chen, J., de Hoogh, K., Gulliver, J., Hoffmann, B., Hertel, O., Ketzel, M., Weinmayr, G., Bauwelinck, M., van Donkelaar, A., Hvidtfeldt, U. A., Atkinson, R., Janssen, N. A. H., Martin, R. V., Samoli, E., Andersen, Z. J., Oftedal, B. M., Stafoggia, M., Bellander, T., Strak, M., Wolf, K., Vienneau, D., Brunekreef, B., and Hoek, G.: Development of Europe-Wide Models for Particle Elemental Composition Using Supervised Linear Regression and Random Forest, Environ. Sci. Technol., 54, 15698–15709, https://doi.org/10.1021/acs.est.0c06595, 2020.
Chen, J., Rodopoulou, S., Hoogh, K. d., Strak, M., Andersen, Z. J., Atkinson, R., Bauwelinck, M., Bellander, T., Brandt, J., Cesaroni, G., Concin, H., Fecht, D., Forastiere, F., Gulliver, J., Hertel, O., Hoffmann, B., Hvidtfeldt, U. A., Janssen, N. A. H., Jöckel, K.-H., Jørgensen, J., Katsouyanni, K., Ketzel, M., Klompmaker, J. O., Lager, A., Leander, K., Liu, S., Ljungman, P., MacDonald, C. J., Magnusson, P. K. E., Mehta, A., Nagel, G., Oftedal, B., Pershagen, G., Peters, A., Raaschou-Nielsen, O., Renzi, M., Rizzuto, D., Samoli, E., Schouw, Y. T. v. d., Schramm, S., Schwarze, P., Sigsgaard, T., Sørensen, M., Stafoggia, M., Tjønneland, A., Vienneau, D., Weinmayr, G., Wolf, K., Brunekreef, B., and Hoek, G.: Long-Term Exposure to Fine Particle Elemental Components and Natural and Cause-Specific Mortality – a Pooled Analysis of Eight European Cohorts within the ELAPSE Project, Environ. Health Perspect., 129, 047009, https://doi.org/10.1289/EHP8368, 2021.
Ciarelli, G., Aksoyoglu, S., El Haddad, I., Bruns, E. A., Crippa, M., Poulain, L., Äijälä, M., Carbone, S., Freney, E., O'Dowd, C., Baltensperger, U., and Prévôt, A. S. H.: Modelling winter organic aerosol at the European scale with CAMx: evaluation and source apportionment with a VBS parameterization based on novel wood burning smog chamber experiments, Atmos. Chem. Phys., 17, 7653–7669, https://doi.org/10.5194/acp-17-7653-2017, 2017.
Coggon, M. M., Gkatzelis, G. I., McDonald, B. C., Gilman, J. B., Schwantes, R. H., Abuhassan, N., Aikin, K. C., Arend, M. F., Berkoff, T. A., Brown, S. S., Campos, T. L., Dickerson, R. R., Gronoff, G., Hurley, J. F., Isaacman-VanWertz, G., Koss, A. R., Li, M., McKeen, S. A., Moshary, F., Peischl, J., Pospisilova, V., Ren, X., Wilson, A., Wu, Y., Trainer, M., and Warneke, C.: Volatile chemical product emissions enhance ozone and modulate urban chemistry, P. Natl. Acad. Sci. USA, 118, e2026653118, https://doi.org/10.1073/pnas.2026653118, 2021.
Cohen, A. J., Brauer, M., Burnett, R., Anderson, H. R., Frostad, J., Estep, K., Balakrishnan, K., Brunekreef, B., Dandona, L., Dandona, R., Feigin, V., Freedman, G., Hubbell, B., Jobling, A., Kan, H., Knibbs, L., Liu, Y., Martin, R., Morawska, L., Pope, C. A., Shin, H., Straif, K., Shaddick, G., Thomas, M., van Dingenen, R., van Donkelaar, A., Vos, T., Murray, C. J. L., and Forouzanfar, M. H.: Estimates and 25-year trends of the global burden of disease attributable to ambient air pollution: an analysis of data from the Global Burden of Diseases Study 2015, The Lancet, 389, 1907–1918, https://doi.org/10.1016/S0140-6736(17)30505-6, 2017.
Crooks, J. L., Cascio, W. E., Percy, M. S., Reyes, J., Neas, L. M., and Hilborn, E. D.: The Association between Dust Storms and Daily Non-Accidental Mortality in the United States, 1993–2005, Environ. Health Perspect., 124, 1735–1743, https://doi.org/10.1289/EHP216, 2016.
Daellenbach, K. R., Stefenelli, G., Bozzetti, C., Vlachou, A., Fermo, P., Gonzalez, R., Piazzalunga, A., Colombi, C., Canonaco, F., Hueglin, C., Kasper-Giebl, A., Jaffrezo, J.-L., Bianchi, F., Slowik, J. G., Baltensperger, U., El-Haddad, I., and Prévôt, A. S. H.: Long-term chemical analysis and organic aerosol source apportionment at nine sites in central Europe: source identification and uncertainty assessment, Atmos. Chem. Phys., 17, 13265–13282, https://doi.org/10.5194/acp-17-13265-2017, 2017.
Daellenbach, K. R., Uzu, G., Jiang, J., Cassagnes, L. E., Leni, Z., Vlachou, A., Stefenelli, G., Canonaco, F., Weber, S., Segers, A., Kuenen, J. J. P., Schaap, M., Favez, O., Albinet, A., Aksoyoglu, S., Dommen, J., Baltensperger, U., Geiser, M., El Haddad, I., Jaffrezo, J. L., and Prévôt, A. S. H.: Sources of particulate-matter air pollution and its oxidative potential in Europe, Nature, 587, 414–419, https://doi.org/10.1038/s41586-020-2902-8, 2020.
David, J. D., Stacey, W. B., Michael, B., Carol, B., Kevin, A. B., Russell, C., Larry Di, G., Michael, J. G., Sina, H., Edward, H., Michael, J., Veljko, J., Olga, V. K., Yang, L., Alexei, I. L., Randall, V. M., Abigail, N., Bart, D. O., Beate, R., Joel, S., Jun, W., and Feng, X.: Advances in multiangle satellite remote sensing of speciated airborne particulate matter and association with adverse health effects: from MISR to MAIA, J. Appl. Remote Sens., 12, 042603, https://doi.org/10.1117/1.JRS.12.042603, 2018.
de Bont, J., Jaganathan, S., Dahlquist, M., Persson, Å., Stafoggia, M., and Ljungman, P.: Ambient air pollution and cardiovascular diseases: An umbrella review of systematic reviews and meta-analyses, J. Int. Med., 291, 779–800, https://doi.org/10.1111/joim.13467, 2022.
Decker, Z. C. J., Zarzana, K. J., Coggon, M., Min, K.-E., Pollack, I., Ryerson, T. B., Peischl, J., Edwards, P., Dubé, W. P., Markovic, M. Z., Roberts, J. M., Veres, P. R., Graus, M., Warneke, C., de Gouw, J., Hatch, L. E., Barsanti, K. C., and Brown, S. S.: Nighttime Chemical Transformation in Biomass Burning Plumes: A Box Model Analysis Initialized with Aircraft Observations, Environ. Sci. Technol., 53, 2529–2538, https://doi.org/10.1021/acs.est.8b05359, 2019.
de Hoogh, K., Wang, M., Adam, M., Badaloni, C., Beelen, R., Birk, M., Cesaroni, G., Cirach, M., Declercq, C., Dëdelë, A., Dons, E., de Nazelle, A., Eeftens, M., Eriksen, K., Eriksson, C., Fischer, P., Graþulevièienë, R., Gryparis, A., Hoffmann, B., Jerrett, M., Katsouyanni, K., Iakovides, M., Lanki, T., Lindley, S., Madsen, C., Mölter, A., Mosler, G., Nádor, G., Nieuwenhuijsen, M., Pershagen, G., Peters, A., Phuleria, H., Probst-Hensch, N., Raaschou-Nielsen, O., Quass, U., Ranzi, A., Stephanou, E., Sugiri, D., Schwarze, P., Tsai, M.-Y., Yli-Tuomi, T., Varró, M. J., Vienneau, D., Weinmayr, G., Brunekreef, B., and Hoek, G.: Development of Land Use Regression Models for Particle Composition in Twenty Study Areas in Europe, Environ. Sci. Technol., 47, 5778–5786, https://doi.org/10.1021/es400156t, 2013.
de Hoogh, K., Gulliver, J., Donkelaar, A. v., Martin, R. V., Marshall, J. D., Bechle, M. J., Cesaroni, G., Pradas, M. C., Dedele, A., Eeftens, M., Forsberg, B., Galassi, C., Heinrich, J., Hoffmann, B., Jacquemin, B., Katsouyanni, K., Korek, M., Künzli, N., Lindley, S. J., Lepeule, J., Meleux, F., de Nazelle, A., Nieuwenhuijsen, M., Nystad, W., Raaschou-Nielsen, O., Peters, A., Peuch, V.-H., Rouil, L., Udvardy, O., Slama, R., Stempfelet, M., Stephanou, E. G., Tsai, M. Y., Yli-Tuomi, T., Weinmayr, G., Brunekreef, B., Vienneau, D., and Hoek, G.: Development of West-European PM2.5 and NO2 land use regression models incorporating satellite-derived and chemical transport modelling data, Environ. Res., 151, 1–10, https://doi.org/10.1016/j.envres.2016.07.005, 2016.
de Hoogh, K., Héritier, H., Stafoggia, M., Künzli, N., and Kloog, I.: Modelling daily PM2.5 concentrations at high spatio-temporal resolution across Switzerland, Environ. Pollut., 233, 1147–1154, https://doi.org/10.1016/j.envpol.2017.10.025, 2018.
Di, Q., Kloog, I., Koutrakis, P., Lyapustin, A., Wang, Y., and Schwartz, J.: Assessing PM2.5 Exposures with High Spatiotemporal Resolution across the Continental United States, Environ. Sci. Technol., 50, 4712–4721, https://doi.org/10.1021/acs.est.5b06121, 2016.
Di, Q., Amini, H., Shi, L., Kloog, I., Silvern, R., Kelly, J., Sabath, M. B., Choirat, C., Koutrakis, P., Lyapustin, A., Wang, Y., Mickley, L. J., and Schwartz, J.: An ensemble-based model of PM2.5 concentration across the contiguous United States with high spatiotemporal resolution, Environ. Int., 130, 104909, https://doi.org/10.1016/j.envint.2019.104909, 2019.
Dockery, D. W., Pope, C. A., Xu, X., Spengler, J. D., Ware, J. H., Fay, M. E., Ferris, B. G., and Speizer, F. E.: An Association between Air Pollution and Mortality in Six U.S. Cities, New Engl. J. Med., 329, 1753–1759, https://doi.org/10.1056/nejm199312093292401, 1993.
Dominici, F., Zanobetti, A., Schwartz, J., Braun, D., Sabath, B., and Wu, X.: Assessing Adverse Health Effects of Long-Term Exposure to Low Levels of Ambient Air Pollution: Implementation of Causal Inference Methods. Res Rep Health Eff Inst. 2022 January, (211), 1-56, PMID: 36193708; PMCID: PMC9530797, 2022.
Eeftens, M., Meier, R., Schindler, C., Aguilera, I., Phuleria, H., Ineichen, A., Davey, M., Ducret-Stich, R., Keidel, D., Probst-Hensch, N., Künzli, N., and Tsai, M.-Y.: Development of land use regression models for nitrogen dioxide, ultrafine particles, lung deposited surface area, and four other markers of particulate matter pollution in the Swiss SAPALDIA regions, Environ. Health, 15, 53, https://doi.org/10.1186/s12940-016-0137-9, 2016.
Eeftens, M., Beelen, R., de Hoogh, K., Bellander, T., Cesaroni, G., Cirach, M., Declercq, C., Dëdelë, A., Dons, E., de Nazelle, A., Dimakopoulou, K., Eriksen, K., Falq, G., Fischer, P., Galassi, C., Graþulevièienë, R., Heinrich, J., Hoffmann, B., Jerrett, M., Keidel, D., Korek, M., Lanki, T., Lindley, S., Madsen, C., Mölter, A., Nádor, G., Nieuwenhuijsen, M., Nonnemacher, M., Pedeli, X., Raaschou-Nielsen, O., Patelarou, E., Quass, U., Ranzi, A., Schindler, C., Stempfelet, M., Stephanou, E., Sugiri, D., Tsai, M. Y., Yli-Tuomi, T., Varró, M. J., Vienneau, D., Klot, S., Wolf, K., Brunekreef, B., and Hoek, G.: Development of Land Use Regression models for PM(2.5), PM(2.5) absorbance, PM(10) and PM(coarse) in 20 European study areas; results of the ESCAPE project, Environ. Sci. Technol., 46, 11195–11205, https://doi.org/10.1021/es301948k, 2012a.
Eeftens, M., Tsai, M.-Y., Ampe, C., Anwander, B., Beelen, R., Bellander, T., Cesaroni, G., Cirach, M., Cyrys, J., de Hoogh, K., De Nazelle, A., de Vocht, F., Declercq, C., Dëdelë, A., Eriksen, K., Galassi, C., Graþulevièienë, R., Grivas, G., Heinrich, J., Hoffmann, B., Iakovides, M., Ineichen, A., Katsouyanni, K., Korek, M., Krämer, U., Kuhlbusch, T., Lanki, T., Madsen, C., Meliefste, K., Mölter, A., Mosler, G., Nieuwenhuijsen, M., Oldenwening, M., Pennanen, A., Probst-Hensch, N., Quass, U., Raaschou-Nielsen, O., Ranzi, A., Stephanou, E., Sugiri, D., Udvardy, O., Vaskövi, É., Weinmayr, G., Brunekreef, B., and Hoek, G.: Spatial variation of PM2.5, PM10, PM2.5 absorbance and PMcoarse concentrations between and within 20 European study areas and the relationship with NO2 – Results of the ESCAPE project, Atmos. Environ., 62, 303–317, https://doi.org/10.1016/j.atmosenv.2012.08.038, 2012b.
Egnér, H. and Eriksson, E.: Current Data on the Chemical Composition of Air and Precipitation, Tellus, 7, 134–139, https://doi.org/10.3402/tellusa.v7i1.8763, 1955.
Elser, M., Bozzetti, C., El-Haddad, I., Maasikmets, M., Teinemaa, E., Richter, R., Wolf, R., Slowik, J. G., Baltensperger, U., and Prévôt, A. S. H.: Urban increments of gaseous and aerosol pollutants and their sources using mobile aerosol mass spectrometry measurements, Atmos. Chem. Phys., 16, 7117–7134, https://doi.org/10.5194/acp-16-7117-2016, 2016.
Elser, M., El-Haddad, I., Maasikmets, M., Bozzetti, C., Wolf, R., Ciarelli, G., Slowik, J. G., Richter, R., Teinemaa, E., Hüglin, C., Baltensperger, U., and Prévôt, A. S. H.: High contributions of vehicular emissions to ammonia in three European cities derived from mobile measurements, Atmos. Environ., 175, 210–220, https://doi.org/10.1016/j.atmosenv.2017.11.030, 2018.
Evelyn, J.: Fumifugium, Or, The Inconveniencie of the Aer and Smoak of London Dissipated: Together with Some Remedies Humbly Proposed, W. Godbid, 1–49 pp., https://play.google.com/store/books/details?id=AeI5NYp7WCwC&rdid=book-AeI5NYp7WCwC&rdot=1&pli=1 (last access: 23 August 2024), 1772.
Fang, T., Guo, H., Zeng, L., Verma, V., Nenes, A., and Weber, R. J.: Highly Acidic Ambient Particles, Soluble Metals, and Oxidative Potential: A Link between Sulfate and Aerosol Toxicity, Environ. Sci. Technol., 51, 2611–2620, https://doi.org/10.1021/acs.est.6b06151, 2017.
Fouladi, F., Bailey, M. J., Patterson, W. B., Sioda, M., Blakley, I. C., Fodor, A. A., Jones, R. B., Chen, Z., Kim, J. S., Lurmann, F., Martino, C., Knight, R., Gilliland, F. D., and Alderete, T. L.: Air pollution exposure is associated with the gut microbiome as revealed by shotgun metagenomic sequencing, Environ. Int., 138, 105604, https://doi.org/10.1016/j.envint.2020.105604, 2020.
Fowler, D., Amann, M., Anderson, R., Ashmore, M., Cox, P., Depledge, M., Derwent, D., Grennfelt, P., Hewitt, N., and Hov, O.: Ground-level ozone in the 21st century: future trends, impacts and policy implications, 1–148 pp., https://royalsociety.org/-/media/policy/publications/2008/7925.pdf (last access: 23 August 2024), 2008.
Fowler, D., Brimblecombe, P., Burrows, J., Heal, M. R., Grennfelt, P., Stevenson, D. S., Jowett, A., Nemitz, E., Coyle, M., Liu, X., Chang, Y., Fuller, G. W., Sutton, M. A., Klimont, Z., Unsworth, M. H., and Vieno, M.: A chronology of global air quality, Pilos. T. R. Soc. A, 378, 20190314, https://doi.org/10.1098/rsta.2019.0314, 2020.
Fröhlich, R., Cubison, M. J., Slowik, J. G., Bukowiecki, N., Prévôt, A. S. H., Baltensperger, U., Schneider, J., Kimmel, J. R., Gonin, M., Rohner, U., Worsnop, D. R., and Jayne, J. T.: The ToF-ACSM: a portable aerosol chemical speciation monitor with TOFMS detection, Atmos. Meas. Tech., 6, 3225–3241, https://doi.org/10.5194/amt-6-3225-2013, 2013.
Fry, J. L., Brown, S. S., Middlebrook, A. M., Edwards, P. M., Campuzano-Jost, P., Day, D. A., Jimenez, J. L., Allen, H. M., Ryerson, T. B., Pollack, I., Graus, M., Warneke, C., de Gouw, J. A., Brock, C. A., Gilman, J., Lerner, B. M., Dubé, W. P., Liao, J., and Welti, A.: Secondary organic aerosol (SOA) yields from NO3 radical + isoprene based on nighttime aircraft power plant plume transects, Atmos. Chem. Phys., 18, 11663–11682, https://doi.org/10.5194/acp-18-11663-2018, 2018.
Furger, M., Minguillón, M. C., Yadav, V., Slowik, J. G., Hüglin, C., Fröhlich, R., Petterson, K., Baltensperger, U., and Prévôt, A. S. H.: Elemental composition of ambient aerosols measured with high temporal resolution using an online XRF spectrometer, Atmos. Meas. Tech., 10, 2061–2076, https://doi.org/10.5194/amt-10-2061-2017, 2017.
Gao, Y., Huang, W., Yu, P., Xu, R., Yang, Z., Gasevic, D., Ye, T., Guo, Y., and Li, S.: Long-term impacts of non-occupational wildfire exposure on human health: A systematic review, Environ. Pollut., 320, 121041, https://doi.org/10.1016/j.envpol.2023.121041, 2023.
Ghidiyal, S. and Dahiya, S.: Tracing the Hazy Air: Progress Report on National Clean Air Programme, 1–47 pp., https://energyandcleanair.org/wp/wp-content/uploads/2022/01/NCAP-Report_Jan22_.pdf (last access: 23 August 2024), 2022.
Gkatzelis, G. I., Coggon, M. M., McDonald, B. C., Peischl, J., Gilman, J. B., Aikin, K. C., Robinson, M. A., Canonaco, F., Prevot, A. S. H., Trainer, M., and Warneke, C.: Observations Confirm that Volatile Chemical Products Are a Major Source of Petrochemical Emissions in U.S. Cities, Environ. Sci. Technol., 55, 4332–4343, https://doi.org/10.1021/acs.est.0c05471, 2021.
Gomez, J., Allen, R. J., Turnock, S. T., Horowitz, L. W., Tsigaridis, K., Bauer, S. E., Olivié, D., Thomson, E. S., and Ginoux, P.: The projected future degradation in air quality is caused by more abundant natural aerosols in a warmer world, Commun. Earth Environ., 4, 22, https://doi.org/10.1038/s43247-023-00688-7, 2023.
Grant, E. and Runkle, J. D.: Long-term health effects of wildfire exposure: A scoping review, The J. Clim. Change Health, 6, 100110, https://doi.org/10.1016/j.joclim.2021.100110, 2022.
Graunt, J.: Natural and political observations made upon the bills of mortality, 2, Johns Hopkins Press, 1–90 pp., https://books.google.ch/books/about/Natural_and_Political_Observations_Made.html?id=j_E-AQAAIAAJ&redir_esc=y (last access: 23 August 2024), 1939.
Griffin, D., Zhao, X., McLinden, C. A., Boersma, F., Bourassa, A., Dammers, E., Degenstein, D., Eskes, H., Fehr, L., Fioletov, V., Hayden, K., Kharol, S. K., Li, S.-M., Makar, P., Martin, R. V., Mihele, C., Mittermeier, R. L., Krotkov, N., Sneep, M., Lamsal, L. N., Linden, M. t., Geffen, J. v., Veefkind, P., and Wolde, M.: High-Resolution Mapping of Nitrogen Dioxide With TROPOMI: First Results and Validation Over the Canadian Oil Sands, 46, 1049–1060, https://doi.org/10.1029/2018GL081095, 2019.
Gu, P., Li, H. Z., Ye, Q., Robinson, E. S., Apte, J. S., Robinson, A. L., and Presto, A. A.: Intracity Variability of Particulate Matter Exposure Is Driven by Carbonaceous Sources and Correlated with Land-Use Variables, Environ. Sci. Technol., 52, 11545–11554, https://doi.org/10.1021/acs.est.8b03833, 2018.
Gunthe, S. S., Liu, P., Panda, U., Raj, S. S., Sharma, A., Darbyshire, E., Reyes-Villegas, E., Allan, J., Chen, Y., Wang, X., Song, S., Pöhlker, M. L., Shi, L., Wang, Y., Kommula, S. M., Liu, T., Ravikrishna, R., McFiggans, G., Mickley, L. J., Martin, S. T., Pöschl, U., Andreae, M. O., and Coe, H.: Enhanced aerosol particle growth sustained by high continental chlorine emission in India, Nat. Geosci., 14, 77–84, https://doi.org/10.1038/s41561-020-00677-x, 2021.
Guo, Y., Wu, Y., Ye, T., Zhang, L., Johnson, A., and Li, S.: A causal modelling framework for short-term effects of PM2.5 on hospitalisations: A nationwide time series study in Brazil, Environ. Int., 171, 107688, https://doi.org/10.1016/j.envint.2022.107688, 2023.
Hallquist, M., Wenger, J. C., Baltensperger, U., Rudich, Y., Simpson, D., Claeys, M., Dommen, J., Donahue, N. M., George, C., Goldstein, A. H., Hamilton, J. F., Herrmann, H., Hoffmann, T., Iinuma, Y., Jang, M., Jenkin, M. E., Jimenez, J. L., Kiendler-Scharr, A., Maenhaut, W., McFiggans, G., Mentel, Th. F., Monod, A., Prévôt, A. S. H., Seinfeld, J. H., Surratt, J. D., Szmigielski, R., and Wildt, J.: The formation, properties and impact of secondary organic aerosol: current and emerging issues, Atmos. Chem. Phys., 9, 5155–5236, https://doi.org/10.5194/acp-9-5155-2009, 2009.
Hammer, M. S., van Donkelaar, A., Li, C., Lyapustin, A., Sayer, A. M., Hsu, N. C., Levy, R. C., Garay, M. J., Kalashnikova, O. V., Kahn, R. A., Brauer, M., Apte, J. S., Henze, D. K., Zhang, L., Zhang, Q., Ford, B., Pierce, J. R., and Martin, R. V.: Global Estimates and Long-Term Trends of Fine Particulate Matter Concentrations (1998–2018), Environ. Sci. Technol., 54, 7879–7890, https://doi.org/10.1021/acs.est.0c01764, 2020.
Hankey, S. and Marshall, J. D.: Land Use Regression Models of On-Road Particulate Air Pollution (Particle Number, Black Carbon, PM2.5, Particle Size) Using Mobile Monitoring, Environ. Sci. Technol., 49, 9194–9202, https://doi.org/10.1021/acs.est.5b01209, 2015.
Heft-Neal, S., Burney, J., Bendavid, E., and Burke, M.: Robust relationship between air quality and infant mortality in Africa, Nature, 559, 254–258, https://doi.org/10.1038/s41586-018-0263-3, 2018.
Hoek, G.: Methods for Assessing Long-Term Exposures to Outdoor Air Pollutants, Curr. Environ. Health Rep., 4, 450–462, https://doi.org/10.1007/s40572-017-0169-5, 2017.
Hoek, G., Beelen, R., Kos, G., Dijkema, M., Zee, S. C. v. d., Fischer, P. H., and Brunekreef, B.: Land Use Regression Model for Ultrafine Particles in Amsterdam, Environ. Sci. Technol., 45, 622–628, https://doi.org/10.1021/es1023042, 2011.
Holtjer, J. C. S., Bloemsma, L. D., Beijers, R. J. H. C. G., Cornelissen, M. E. B., Hilvering, B., Houweling, L., Vermeulen, R. C. H., Downward, G. S., and Zee, A.-H. M.-V. d.: Identifying risk factors for COPD and adult-onset asthma: an umbrella review, Eur. Respir. Rev., 32, 230009, https://doi.org/10.1183/16000617.0009-2023, 2023.
Hu, X., Belle, J. H., Meng, X., Wildani, A., Waller, L. A., Strickland, M. J., and Liu, Y.: Estimating PM2.5 Concentrations in the Conterminous United States Using the Random Forest Approach, Environ. Sci. Technol., 51, 6936–6944, https://doi.org/10.1021/acs.est.7b01210, 2017.
Huang, K., Xiao, Q., Meng, X., Geng, G., Wang, Y., Lyapustin, A., Gu, D., and Liu, Y.: Predicting monthly high-resolution PM2.5 concentrations with random forest model in the North China Plain, Environ. Pollut., 242, 675–683, https://doi.org/10.1016/j.envpol.2018.07.016, 2018.
Huang, R.-J., Zhang, Y., Bozzetti, C., Ho, K.-F., Cao, J.-J., Han, Y., Daellenbach, K. R., Slowik, J. G., Platt, S. M., Canonaco, F., Zotter, P., Wolf, R., Pieber, S. M., Bruns, E. A., Crippa, M., Ciarelli, G., Piazzalunga, A., Schwikowski, M., Abbaszade, G., Schnelle-Kreis, J., Zimmermann, R., An, Z., Szidat, S., Baltensperger, U., Haddad, I. E., and Prévôt, A. S. H.: High secondary aerosol contribution to particulate pollution during haze events in China, Nature, 514, 218–222, https://doi.org/10.1038/nature13774, 2014.
Hyland, A., Travers, M. J., Dresler, C., Higbee, C., and Cummings, K. M.: A 32-country comparison of tobacco smoke derived particle levels in indoor public places, Tob. Control, 17, 159, https://doi.org/10.1136/tc.2007.020479, 2008.
Jedynska, A., Hoek, G., Wang, M., Eeftens, M., Cyrys, J., Keuken, M., Ampe, C., Beelen, R., Cesaroni, G., Forastiere, F., Cirach, M., de Hoogh, K., De Nazelle, A., Nystad, W., Declercq, C., Eriksen, K. T., Dimakopoulou, K., Lanki, T., Meliefste, K., Nieuwenhuijsen, M. J., Yli-Tuomi, T., Raaschou-Nielsen, O., Brunekreef, B., and Kooter, I. M.: Development of Land Use Regression Models for Elemental, Organic Carbon, PAH, and Hopanes/Steranes in 10 ESCAPE/TRANSPHORM European Study Areas, Environ. Sci. Technol., 48, 14435–14444, https://doi.org/10.1021/es502568z, 2014.
Jedynska, A., Hoek, G., Wang, M., Eeftens, M., Cyrys, J., Beelen, R., Cirach, M., De Nazelle, A., Keuken, M., Visschedijk, A., Nystad, W., Akhlaghi, H. M., Meliefste, K., Nieuwenhuijsen, M., de Hoogh, K., Brunekreef, B., and Kooter, I. M.: Spatial variations of levoglucosan in four European study areas, Sci. Total Environ., 505, 1072–1081, https://doi.org/10.1016/j.scitotenv.2014.10.091, 2015.
Jerrett, M., Arain, A., Kanaroglou, P., Beckerman, B., Potoglou, D., Sahsuvaroglu, T., Morrison, J., and Giovis, C.: A review and evaluation of intraurban air pollution exposure models, J. Expo. Sci. Env. Epid., 15, 185–204, https://doi.org/10.1038/sj.jea.7500388, 2005.
Jiang, H., Bao, F., Wang, J., Chen, J., Zhu, Y., Huang, D., Chen, C., and Zhao, J.: Direct Formation of Electronic Excited NO2 Contributes to the High Yield of HONO during Photosensitized Renoxification, Environ. Sci. Technol., 57, 11144–11151, https://doi.org/10.1021/acs.est.3c01342, 2023.
Jiang, J., Aksoyoglu, S., El-Haddad, I., Ciarelli, G., Denier van der Gon, H. A. C., Canonaco, F., Gilardoni, S., Paglione, M., Minguillón, M. C., Favez, O., Zhang, Y., Marchand, N., Hao, L., Virtanen, A., Florou, K., O'Dowd, C., Ovadnevaite, J., Baltensperger, U., and Prévôt, A. S. H.: Sources of organic aerosols in Europe: a modeling study using CAMx with modified volatility basis set scheme, Atmos. Chem. Phys., 19, 15247–15270, https://doi.org/10.5194/acp-19-15247-2019, 2019.
Jimenez, J. L., Canagaratna, M. R., Donahue, N. M., Prevot, A. S. H., Zhang, Q., Kroll, J. H., DeCarlo, P. F., Allan, J. D., Coe, H., Ng, N. L., Aiken, A. C., Docherty, K. S., Ulbrich, I. M., Grieshop, A. P., Robinson, A. L., Duplissy, J., Smith, J. D., Wilson, K. R., Lanz, V. A., Hueglin, C., Sun, Y. L., Tian, J., Laaksonen, A., Raatikainen, T., Rautiainen, J., Vaattovaara, P., Ehn, M., Kulmala, M., Tomlinson, J. M., Collins, D. R., Cubison, M. J., Dunlea, J., Huffman, J. A., Onasch, T. B., Alfarra, M. R., Williams, P. I., Bower, K., Kondo, Y., Schneider, J., Drewnick, F., Borrmann, S., Weimer, S., Demerjian, K., Salcedo, D., Cottrell, L., Griffin, R., Takami, A., Miyoshi, T., Hatakeyama, S., Shimono, A., Sun, J. Y., Zhang, Y. M., Dzepina, K., Kimmel, J. R., Sueper, D., Jayne, J. T., Herndon, S. C., Trimborn, A. M., Williams, L. R., Wood, E. C., Middlebrook, A. M., Kolb, C. E., Baltensperger, U., and Worsnop, D. R.: Evolution of Organic Aerosols in the Atmosphere, Science, 326, 1525, https://doi.org/10.1126/science.1180353, 2009.
Seinfeld, J. H. and Pandis, S. N.: : Atmospheric Chemistry and Physics: From Air Pollution to Climate Change, 3rd Edition, Wiley, 1–1152 pp., https://www.wiley.com/en-us/Atmospheric+Chemistry+and+Physics +From+Air+Pollution+to+Climate+Change +3rd+Edition-p-9781118947401 (last access: 23 August 2024), 2016.
Jones, W. H. S., Withington, E. T., Potter, P., and Smith, W. D.: Hippocrates Volume II# 148, Harvard University Press, 1–416 pp., https://books.google.ch/books/about/Hippocrates_Volume_II_148.html?id=GSRJAAAAYAAJ&redir_esc=y (last access: 23 August 2024), 1923.
Kim, S.-Y., Sheppard, L., Bergen, S., Szpiro, A. A., Sampson, P. D., Kaufman, J. D., and Vedal, S.: Prediction of fine particulate matter chemical components with a spatio-temporal model for the Multi-Ethnic Study of Atherosclerosis cohort, J. Expo. Sci. Env. Epid., 26, 520–528, https://doi.org/10.1038/jes.2016.29, 2016.
Klein, F., Baltensperger, U., Prévôt, A. S. H., and El Haddad, I.: Quantification of the impact of cooking processes on indoor concentrations of volatile organic species and primary and secondary organic aerosols, Indoor Air, 29, 926–942, https://doi.org/10.1111/ina.12597, 2019.
Kodros, J. K., Papanastasiou, D. K., Paglione, M., Masiol, M., Squizzato, S., Florou, K., Skyllakou, K., Kaltsonoudis, C., Nenes, A., and Pandis, S. N.: Rapid dark aging of biomass burning as an overlooked source of oxidized organic aerosol, P. Natl. Acad. Sci. USA, 117, 33028–33033, https://doi.org/10.1073/pnas.2010365117, 2020.
Korsiak, J., Pinault, L., Christidis, T., Burnett, R. T., Abrahamowicz, M., and Weichenthal, S.: Long-term exposure to wildfires and cancer incidence in Canada: a population-based observational cohort study, The Lancet Planet. Health, 6, e400–e409, https://doi.org/10.1016/S2542-5196(22)00067-5, 2022.
Kukutschová, J. and Filip, P.: Chapter 6 – Review of Brake Wear Emissions: A Review of Brake Emission Measurement Studies: Identification of Gaps and Future Needs, in: Non-Exhaust Emissions, edited by: Amato, F., Academic Press, 123–146, https://doi.org/10.1016/B978-0-12-811770-5.00006-6, 2018.
Kulmala, M., Dada, L., Daellenbach, K. R., Yan, C., Stolzenburg, D., Kontkanen, J., Ezhova, E., Hakala, S., Tuovinen, S., Kokkonen, T. V., Kurppa, M., Cai, R., Zhou, Y., Yin, R., Baalbaki, R., Chan, T., Chu, B., Deng, C., Fu, Y., Ge, M., He, H., Heikkinen, L., Junninen, H., Liu, Y., Lu, Y., Nie, W., Rusanen, A., Vakkari, V., Wang, Y., Yang, G., Yao, L., Zheng, J., Kujansuu, J., Kangasluoma, J., Petäjä, T., Paasonen, P., Järvi, L., Worsnop, D., Ding, A., Liu, Y., Wang, L., Jiang, J., Bianchi, F., and Kerminen, V.-M.: Is reducing new particle formation a plausible solution to mitigate particulate air pollution in Beijing and other Chinese megacities?, Faraday Discuss., 226, 334-347, https://doi.org/10.1039/D0FD00078G, 2021.
Kumar, V., Giannoukos, S., Haslett, S. L., Tong, Y., Singh, A., Bertrand, A., Lee, C. P., Wang, D. S., Bhattu, D., Stefenelli, G., Dave, J. S., Puthussery, J. V., Qi, L., Vats, P., Rai, P., Casotto, R., Satish, R., Mishra, S., Pospisilova, V., Mohr, C., Bell, D. M., Ganguly, D., Verma, V., Rastogi, N., Baltensperger, U., Tripathi, S. N., Prévôt, A. S. H., and Slowik, J. G.: Highly time-resolved chemical speciation and source apportionment of organic aerosol components in Delhi, India, using extractive electrospray ionization mass spectrometry, Atmos. Chem. Phys., 22, 7739–7761, https://doi.org/10.5194/acp-22-7739-2022, 2022.
Landrigan, P. J., Fuller, R., Acosta, N. J. R., Adeyi, O., Arnold, R., Basu, N., Baldé, A. B., Bertollini, R., Bose-O'Reilly, S., Boufford, J. I., Breysse, P. N., Chiles, T., Mahidol, C., Coll-Seck, A. M., Cropper, M. L., Fobil, J., Fuster, V., Greenstone, M., Haines, A., Hanrahan, D., Hunter, D., Khare, M., Krupnick, A., Lanphear, B., Lohani, B., Martin, K., Mathiasen, K. V., McTeer, M. A., Murray, C. J. L., Ndahimananjara, J. D., Perera, F., Potoènik, J., Preker, A. S., Ramesh, J., Rockström, J., Salinas, C., Samson, L. D., Sandilya, K., Sly, P. D., Smith, K. R., Steiner, A., Stewart, R. B., Suk, W. A., van Schayck, O. C. P., Yadama, G. N., Yumkella, K., and Zhong, M.: The Lancet Commission on pollution and health, The Lancet, 391, 462–512, https://doi.org/10.1016/S0140-6736(17)32345-0, 2018.
Le, T., Wang, Y., Liu, L., Yang, J., Yung, Y. L., Li, G., and Seinfeld, J. H.: Unexpected air pollution with marked emission reductions during the COVID-19 outbreak in China, Science, 369, 702–706, https://doi.org/10.1126/science.abb7431, 2020.
Lelieveld, J., Evans, J. S., Fnais, M., Giannadaki, D., and Pozzer, A.: The contribution of outdoor air pollution sources to premature mortality on a global scale, Nature, 525, 367–371, https://doi.org/10.1038/nature15371, 2015.
Lelieveld, J., Klingmüller, K., Pozzer, A., Pöschl, U., Fnais, M., Daiber, A., and Münzel, T.: Cardiovascular disease burden from ambient air pollution in Europe reassessed using novel hazard ratio functions, Eur. Heart J., 40, 1590–1596, https://doi.org/10.1093/eurheartj/ehz135, 2019.
Leni, Z., Cassagnes, L. E., Daellenbach, K. R., El Haddad, I., Vlachou, A., Uzu, G., Prévôt, A. S. H., Jaffrezo, J.-L., Baumlin, N., Salathe, M., Baltensperger, U., Dommen, J., and Geiser, M.: Oxidative stress-induced inflammation in susceptible airways by anthropogenic aerosol, PLOS ONE, 15, e0233425, https://doi.org/10.1371/journal.pone.0233425, 2020.
Li, H. Z., Dallmann, T. R., Gu, P., and Presto, A. A.: Application of mobile sampling to investigate spatial variation in fine particle composition, Atmos. Environ., 142, 71–82, https://doi.org/10.1016/j.atmosenv.2016.07.042, 2016.
Li, K., Jacob, D. J., Liao, H., Zhu, J., Shah, V., Shen, L., Bates, K. H., Zhang, Q., and Zhai, S.: A two-pollutant strategy for improving ozone and particulate air quality in China, Nat. Geosci., 12, 906–910, https://doi.org/10.1038/s41561-019-0464-x, 2019.
Li, W., Zhou, S., Wang, X., Xu, Z., Yuan, C., Yu, Y., Zhang, Q., and Wang, W.: Integrated evaluation of aerosols from regional brown hazes over northern China in winter: Concentrations, sources, transformation, and mixing states, J. Geophys. Res.-Atmos., 116, D09301, https://doi.org/10.1029/2010JD015099, 2011.
Li, W., Shao, L., Zhang, D., Ro, C.-U., Hu, M., Bi, X., Geng, H., Matsuki, A., Niu, H., and Chen, J.: A review of single aerosol particle studies in the atmosphere of East Asia: morphology, mixing state, source, and heterogeneous reactions, J. Cleaner Prod., 112, 1330–1349, https://doi.org/10.1016/j.jclepro.2015.04.050, 2016.
Liu, C., Chen, R., Sera, F., Vicedo-Cabrera, A. M., Guo, Y., Tong, S., Coelho, M. S. Z. S., Saldiva, P. H. N., Lavigne, E., Matus, P., Valdes Ortega, N., Osorio Garcia, S., Pascal, M., Stafoggia, M., Scortichini, M., Hashizume, M., Honda, Y., Hurtado-Díaz, M., Cruz, J., Nunes, B., Teixeira, J. P., Kim, H., Tobias, A., Íñiguez, C., Forsberg, B., Åström, C., Ragettli, M. S., Guo, Y.-L., Chen, B.-Y., Bell, M. L., Wright, C. Y., Scovronick, N., Garland, R. M., Milojevic, A., Kyselý, J., Urban, A., Orru, H., Indermitte, E., Jaakkola, J. J. K., Ryti, N. R. I., Katsouyanni, K., Analitis, A., Zanobetti, A., Schwartz, J., Chen, J., Wu, T., Cohen, A., Gasparrini, A., and Kan, H.: Ambient Particulate Air Pollution and Daily Mortality in 652 Cities, New England J. Med., 381, 705–715, https://doi.org/10.1056/NEJMoa1817364, 2019.
Liu, T., Jiang, Y., Hu, J., Li, Z., Li, X., Xiao, J., Yuan, L., He, G., Zeng, W., Rong, Z., Zhu, S., Ma, W., and Wang, Y.: Joint Associations of Short-Term Exposure to Ambient Air Pollutants with Hospital Admission of Ischemic Stroke, Epidemiology, 34, 282–292, https://doi.org/10.1097/ede.0000000000001581, 2023.
Liu-Kang, C., Gallimore, P. J., Liu, T., and Abbatt, J. P. D.: Photoreaction of biomass burning brown carbon aerosol particles, Environ. Sci.-Atmos., 2, 270–278, https://doi.org/10.1039/D1EA00088H, 2022.
Lv, S., Wu, C., Wang, F., Liu, X., Zhang, S., Chen, Y., Zhang, F., Yang, Y., Wang, H., Huang, C., Fu, Q., Duan, Y., and Wang, G.: Nitrate-Enhanced Gas-to-Particle-Phase Partitioning of Water-Soluble Organic Compounds in Chinese Urban Atmosphere: Implications for Secondary Organic Aerosol Formation, Environ. Sci. Technol. Lett., 10, 14–20, https://doi.org/10.1021/acs.estlett.2c00894, 2023.
Maher, B. A., Ahmed, I. A. M., Karloukovski, V., MacLaren, D. A., Foulds, P. G., Allsop, D., Mann, D. M. A., Torres-Jardón, R., and Calderon-Garciduenas, L.: Magnetite pollution nanoparticles in the human brain, P. Natl. Acad. Sci. USA, 113, 10797, https://doi.org/10.1073/pnas.1605941113, 2016.
Manousakas, M., Furger, M., Daellenbach, K. R., Canonaco, F., Chen, G., Tobler, A., Rai, P., Qi, L., Tremper, A. H., Green, D., Hueglin, C., Slowik, J. G., El Haddad, I., and Prevot, A. S. H.: Source identification of the elemental fraction of particulate matter using size segregated, highly time-resolved data and an optimized source apportionment approach, Atmos. Environ. X, 14, 100165, https://doi.org/10.1016/j.aeaoa.2022.100165, 2022.
Masselot, P., Sera, F., Schneider, R., Kan, H., Lavigne, É., Stafoggia, M., Tobias, A., Chen, H., Burnett, R. T., Schwartz, J., Zanobetti, A., Bell, M. L., Chen, B.-Y., Guo, Y.-L. L., Ragettli, M. S., Vicedo-Cabrera, A. M., Åström, C., Forsberg, B., Íñiguez, C., Garland, R. M., Scovronick, N., Madureira, J., Nunes, B., De la Cruz Valencia, C., Hurtado Diaz, M., Honda, Y., Hashizume, M., Ng, C. F. C., Samoli, E., Katsouyanni, K., Schneider, A., Breitner, S., Ryti, N. R. I., Jaakkola, J. J. K., Maasikmets, M., Orru, H., Guo, Y., Valdés Ortega, N., Matus Correa, P., Tong, S., and Gasparrini, A.: Differential Mortality Risks Associated With PM2.5 Components: A Multi-Country, Multi-City Study, Epidemiology, 33, 167–175, https://doi.org/10.1097/ede.0000000000001455, 2022.
McDonald, B. C., de Gouw, J. A., Gilman, J. B., Jathar, S. H., Akherati, A., Cappa, C. D., Jimenez, J. L., Lee-Taylor, J., Hayes, P. L., McKeen, S. A., Cui, Y. Y., Kim, S.-W., Gentner, D. R., Isaacman-VanWertz, G., Goldstein, A. H., Harley, R. A., Frost, G. J., Roberts, J. M., Ryerson, T. B., and Trainer, M.: Volatile chemical products emerging as largest petrochemical source of urban organic emissions, Science, 359, 760–764, https://doi.org/10.1126/science.aaq0524, 2018.
McDuffie, E. E., Martin, R. V., Spadaro, J. V., Burnett, R., Smith, S. J., O'Rourke, P., Hammer, M. S., van Donkelaar, A., Bindle, L., Shah, V., Jaeglé, L., Luo, G., Yu, F., Adeniran, J. A., Lin, J., and Brauer, M.: Source sector and fuel contributions to ambient PM2.5 and attributable mortality across multiple spatial scales, Nat. Commun., 12, 3594, https://doi.org/10.1038/s41467-021-23853-y, 2021.
Miller, D. J., Actkinson, B., Padilla, L., Griffin, R. J., Moore, K., Lewis, P. G. T., Gardner-Frolick, R., Craft, E., Portier, C. J., Hamburg, S. P., and Alvarez, R. A.: Characterizing Elevated Urban Air Pollutant Spatial Patterns with Mobile Monitoring in Houston, Texas, Environ. Sci. Technol., 54, 2133–2142, https://doi.org/10.1021/acs.est.9b05523, 2020.
Mishra, S., Tripathi, S. N., Kanawade, V. P., Haslett, S. L., Dada, L., Ciarelli, G., Kumar, V., Singh, A., Bhattu, D., Rastogi, N., Daellenbach, K. R., Ganguly, D., Gargava, P., Slowik, J. G., Kulmala, M., Mohr, C., El-Haddad, I., and Prevot, A. S. H.: Rapid night-time nanoparticle growth in Delhi driven by biomass-burning emissions, Nat. Geosci., 16, 224–230, https://doi.org/10.1038/s41561-023-01138-x, 2023.
Mohr, C., Richter, R., DeCarlo, P. F., Prévôt, A. S. H., and Baltensperger, U.: Spatial variation of chemical composition and sources of submicron aerosol in Zurich during wintertime using mobile aerosol mass spectrometer data, Atmos. Chem. Phys., 11, 7465–7482, https://doi.org/10.5194/acp-11-7465-2011, 2011.
Morgan, W. T., Allan, J. D., Bauguitte, S., Darbyshire, E., Flynn, M. J., Lee, J., Liu, D., Johnson, B., Haywood, J., Longo, K. M., Artaxo, P. E., and Coe, H.: Transformation and ageing of biomass burning carbonaceous aerosol over tropical South America from aircraft in situ measurements during SAMBBA, Atmos. Chem. Phys., 20, 5309–5326, https://doi.org/10.5194/acp-20-5309-2020, 2020.
Morton Lippmann Lung, C. C. T. G. K. I. G. D. T.: National Particle Component Toxicity (NPACT) Initiative: Integrated Epidemiologic and Toxicologic Studies of the Health Effects of Particulate Matter Components, Health effect institute, HEI – research report, 177, 1-307, 2023.
Mudway, I. S., Kelly, F. J., and Holgate, S. T.: Oxidative stress in air pollution research, Free Radical Bio. Med., 151, 2–6, https://doi.org/10.1016/j.freeradbiomed.2020.04.031, 2020.
Neira, M. and Prüss-Ustün, A.: Preventing disease through healthy environments: A global assessment of the environmental burden of disease, Toxicol. Lett., 259, S1, https://doi.org/10.1016/j.toxlet.2016.07.028, 2016.
Ng, N. L., Herndon, S. C., Trimborn, A., Canagaratna, M. R., Croteau, P. L., Onasch, T. B., Sueper, D., Worsnop, D. R., Zhang, Q., Sun, Y. L., and Jayne, J. T.: An Aerosol Chemical Speciation Monitor (ACSM) for Routine Monitoring of the Composition and Mass Concentrations of Ambient Aerosol, Aerosol Sci. Technol., 45, 780–794, https://doi.org/10.1080/02786826.2011.560211, 2011.
Nhung, N. T. T., Amini, H., Schindler, C., Kutlar Joss, M., Dien, T. M., Probst-Hensch, N., Perez, L., and Künzli, N.: Short-term association between ambient air pollution and pneumonia in children: A systematic review and meta-analysis of time-series and case-crossover studies, Environ. Pollut., 230, 1000–1008, https://doi.org/10.1016/j.envpol.2017.07.063, 2017.
Nyadanu, S. D., Dunne, J., Tessema, G. A., Mullins, B., Kumi-Boateng, B., Lee Bell, M., Duko, B., and Pereira, G.: Prenatal exposure to ambient air pollution and adverse birth outcomes: An umbrella review of 36 systematic reviews and meta-analyses, Environ. Pollut., 306, 119465, https://doi.org/10.1016/j.envpol.2022.119465, 2022.
Oney, B., Henne, S., Gruber, N., Leuenberger, M., Bamberger, I., Eugster, W., and Brunner, D.: The CarboCount CH sites: characterization of a dense greenhouse gas observation network, Atmos. Chem. Phys., 15, 11147–11164, https://doi.org/10.5194/acp-15-11147-2015, 2015.
Paciorek, C. J., Liu, Y., Moreno-Macias, H., and Kondragunta, S.: Spatiotemporal Associations between GOES Aerosol Optical Depth Retrievals and Ground-Level PM2.5, Environ. Sci. Technol., 42, 5800–5806, https://doi.org/10.1021/es703181j, 2008.
Pai, S. J., Carter, T. S., Heald, C. L., and Kroll, J. H.: Updated World Health Organization Air Quality Guidelines Highlight the Importance of Non-anthropogenic PM2.5, Environ. Sci. Technol. Lett., 9, 501–506, https://doi.org/10.1021/acs.estlett.2c00203, 2022.
Pennington, E. A., Seltzer, K. M., Murphy, B. N., Qin, M., Seinfeld, J. H., and Pye, H. O. T.: Modeling secondary organic aerosol formation from volatile chemical products, Atmos. Chem. Phys., 21, 18247–18261, https://doi.org/10.5194/acp-21-18247-2021, 2021.
Peters, A., Nawrot, T. S., and Baccarelli, A. A.: Hallmarks of environmental insults, Cell, 184, 1455–1468, https://doi.org/10.1016/j.cell.2021.01.043, 2021.
Peters, A., Peters, A., Greiser, K. H., Göttlicher, S., Ahrens, W., Albrecht, M., Bamberg, F., Bärnighausen, T., Becher, H., Berger, K., Beule, A., Boeing, H., Bohn, B., Bohnert, K., Braun, B., Brenner, H., Bülow, R., Castell, S., Damms-Machado, A., Dörr, M., Ebert, N., Ecker, M., Emmel, C., Fischer, B., Franzke, C.-W., Gastell, S., Giani, G., Günther, M., Günther, K., Günther, K.-P., Haerting, J., Haug, U., Heid, I. M., Heier, M., Heinemeyer, D., Hendel, T., Herbolsheimer, F., Hirsch, J., Hoffmann, W., Holleczek, B., Hölling, H., Hörlein, A., Jöckel, K.-H., Kaaks, R., Karch, A., Karrasch, S., Kartschmit, N., Kauczor, H.-U., Keil, T., Kemmling, Y., Klee, B., Klüppelholz, B., Kluttig, A., Kofink, L., Köttgen, A., Kraft, D., Krause, G., Kretz, L., Krist, L., Kühnisch, J., Kuß, O., Legath, N., Lehnich, A.-T., Leitzmann, M., Lieb, W., Linseisen, J., Loeffler, M., Macdonald, A., Maier-Hein, K. H., Mangold, N., Meinke-Franze, C., Meisinger, C., Melzer, J., Mergarten, B., Michels, K. B., Mikolajczyk, R., Moebus, S., Mueller, U., Nauck, M., Niendorf, T., Nikolaou, K., Obi, N., Ostrzinski, S., Panreck, L., Pigeot, I., Pischon, T., Pschibul-Thamm, I., Rathmann, W., Reineke, A., Roloff, S., Rujescu, D., Rupf, S., Sander, O., Schikowski, T., Schipf, S., Schirmacher, P., Schlett, C. L., Schmidt, B., Schmidt, G., Schmidt, M., Schöne, G., Schulz, H., Schulze, M. B., Schweig, A., Sedlmeier, A. M., Selder, S., Six-Merker, J., Sowade, R., Stang, A., Stegle, O., Steindorf, K., Stübs, G., Swart, E., Teismann, H., Thiele, I., Thierry, S., Ueffing, M., Völzke, H., Waniek, S., Weber, A., Werner, N., Wichmann, H. E., Willich, S. N., Wirkner, K., Wolf, K., Wolff, R., Zeeb, H., Zinkhan, M., Zschocke, J., and German National Cohort, C.: Framework and baseline examination of the German National Cohort (NAKO), Eur. J. Epid., 37, 1107–1124, https://doi.org/10.1007/s10654-022-00890-5, 2022.
Pinault, L., Tjepkema, M., Crouse, D. L., Weichenthal, S., van Donkelaar, A., Martin, R. V., Brauer, M., Chen, H., and Burnett, R. T.: Risk estimates of mortality attributed to low concentrations of ambient fine particulate matter in the Canadian community health survey cohort, Environ. Health, 15, 18, https://doi.org/10.1186/s12940-016-0111-6, 2016.
Pope III, C. A., Burnett, R. T., Thun, M. J., Calle, E. E., Krewski, D., Ito, K., and Thurston, G. D.: Lung Cancer, Cardiopulmonary Mortality, and Long-term Exposure to Fine Particulate Air Pollution, JAMA, 287, 1132–1141, https://doi.org/10.1001/jama.287.9.1132, 2002.
Popoola, O. A. M., Carruthers, D., Lad, C., Bright, V. B., Mead, M. I., Stettler, M. E. J., Saffell, J. R., and Jones, R. L.: Use of networks of low cost air quality sensors to quantify air quality in urban settings, Atmos. Environ., 194, 58–70, https://doi.org/10.1016/j.atmosenv.2018.09.030, 2018.
Prince, M., Guerchet, M., and Prina, M.: The Global Impact of Dementia, 2013–2050, https://www.alzint.org/u/2020/08/GlobalImpactDementia2013.pdf (last access: 23 August 2024), 2013.
Probst-Hensch, N., Bochud, M., Chiolero, A., Crivelli, L., Dratva, J., Flahault, A., Frey, D., Kuenzli, N., Puhan, M., Suggs, L. S., and Wirth, C.: Swiss Cohort & Biobank – The White Paper, Public Health Rev., 43, https://doi.org/10.3389/phrs.2022.1605660, 2022.
Pye, H. O. T., D'Ambro, E. L., Lee, B. H., Schobesberger, S., Takeuchi, M., Zhao, Y., Lopez-Hilfiker, F., Liu, J., Shilling, J. E., Xing, J., Mathur, R., Middlebrook, A. M., Liao, J., Welti, A., Graus, M., Warneke, C., de Gouw, J. A., Holloway, J. S., Ryerson, T. B., Pollack, I. B., and Thornton, J. A.: Anthropogenic enhancements to production of highly oxygenated molecules from autoxidation, P. Natl. Acad. Sci. USA, 116, 6641–6646, https://doi.org/10.1073/pnas.1810774116, 2019.
Pye, H. O. T., Ward-Caviness, C. K., Murphy, B. N., Appel, K. W., and Seltzer, K. M.: Secondary organic aerosol association with cardiorespiratory disease mortality in the United States, Nat. Commun., 12, 7215, https://doi.org/10.1038/s41467-021-27484-1, 2021.
Rai, P., Furger, M., El Haddad, I., Kumar, V., Wang, L., Singh, A., Dixit, K., Bhattu, D., Petit, J.-E., Ganguly, D., Rastogi, N., Baltensperger, U., Tripathi, S. N., Slowik, J. G., and Prévôt, A. S. H.: Real-time measurement and source apportionment of elements in Delhi's atmosphere, Sci. Total Environ., 742, 140332, https://doi.org/10.1016/j.scitotenv.2020.140332, 2020.
Rai, P., Slowik, J. G., Furger, M., El Haddad, I., Visser, S., Tong, Y., Singh, A., Wehrle, G., Kumar, V., Tobler, A. K., Bhattu, D., Wang, L., Ganguly, D., Rastogi, N., Huang, R.-J., Necki, J., Cao, J., Tripathi, S. N., Baltensperger, U., and Prévôt, A. S. H.: Highly time-resolved measurements of element concentrations in PM10 and PM2.5: comparison of Delhi, Beijing, London, and Krakow, Atmos. Chem. Phys., 21, 717–730, https://doi.org/10.5194/acp-21-717-2021, 2021.
Requia, W. J., Adams, M. D., Arain, A., Papatheodorou, S., Koutrakis, P., and Mahmoud, M.: Global Association of Air Pollution and Cardiorespiratory Diseases: A Systematic Review, Meta-Analysis, and Investigation of Modifier Variables, Am. J. Public Health, 108, S123–S130, https://doi.org/10.2105/AJPH.2017.303839, 2017.
Rohr, A. C. and Wyzga, R. E.: Attributing health effects to individual particulate matter constituents, Atmos. Environ., 62, 130–152, https://doi.org/10.1016/j.atmosenv.2012.07.036, 2012.
Schweizer, C., Edwards, R. D., Bayer-Oglesby, L., Gauderman, W. J., Ilacqua, V., Juhani Jantunen, M., Lai, H. K., Nieuwenhuijsen, M., and Künzli, N.: Indoor time–microenvironment–activity patterns in seven regions of Europe, J. Exp. Sci. Environ. Epidemiol., 17, 170–181, https://doi.org/10.1038/sj.jes.7500490, 2007.
Seinfeld, J. H. and Pandis, S. N.: Atmospheric Chemistry and Physics: From Air Pollution to Climate Change, Wiley, 207–214, https://doi.org/10.1097/ede.0000000000000614, 2016.
Shah, R. U., Robinson, E. S., Gu, P., Robinson, A. L., Apte, J. S., and Presto, A. A.: High-spatial-resolution mapping and source apportionment of aerosol composition in Oakland, California, using mobile aerosol mass spectrometry, Atmos. Chem. Phys., 18, 16325–16344, https://doi.org/10.5194/acp-18-16325-2018, 2018.
Shah, R. U., Coggon, M. M., Gkatzelis, G. I., McDonald, B. C., Tasoglou, A., Huber, H., Gilman, J., Warneke, C., Robinson, A. L., and Presto, A. A.: Urban Oxidation Flow Reactor Measurements Reveal Significant Secondary Organic Aerosol Contributions from Volatile Emissions of Emerging Importance, Environ. Sci. Technol., 54, 714–725, https://doi.org/10.1021/acs.est.9b06531, 2020.
Shairsingh, K. K., Jeong, C.-H., Wang, J. M., and Evans, G. J.: Characterizing the spatial variability of local and background concentration signals for air pollution at the neighbourhood scale, Atmos. Environ., 183, 57–68, https://doi.org/10.1016/j.atmosenv.2018.04.010, 2018.
Shen, Y., de Hoogh, K., Schmitz, O., Clinton, N., Tuxen-Bettman, K., Brandt, J., Christensen, J. H., Frohn, L. M., Geels, C., Karssenberg, D., Vermeulen, R., and Hoek, G.: Europe-wide air pollution modeling from 2000 to 2019 using geographically weighted regression, Environ. Int., 168, 107485, https://doi.org/10.1016/j.envint.2022.107485, 2022.
Shi, L., Wu, X., Danesh Yazdi, M., Braun, D., Abu Awad, Y., Wei, Y., Liu, P., Di, Q., Wang, Y., Schwartz, J., Dominici, F., Kioumourtzoglou, M.-A., and Zanobetti, A.: Long-term effects of PM2.5 on neurological disorders in the American Medicare population: a longitudinal cohort study, The Lancet Planet. Health, 4, e557–e565, https://doi.org/10.1016/S2542-5196(20)30227-8, 2020.
Shi, L., Zhu, Q., Wang, Y., Hao, H., Zhang, H., Schwartz, J., Amini, H., van Donkelaar, A., Martin, R. V., Steenland, K., Sarnat, J. A., Caudle, W. M., Ma, T., Li, H., Chang, H. H., Liu, J. Z., Wingo, T., Mao, X., Russell, A. G., Weber, R. J., and Liu, P.: Incident dementia and long-term exposure to constituents of fine particle air pollution: A national cohort study in the United States, P. Natl. Acad. Sci. USA, 120, e2211282119, https://doi.org/10.1073/pnas.2211282119, 2023.
Shrivastava, M., Andreae, M. O., Artaxo, P., Barbosa, H. M. J., Berg, L. K., Brito, J., Ching, J., Easter, R. C., Fan, J., Fast, J. D., Feng, Z., Fuentes, J. D., Glasius, M., Goldstein, A. H., Alves, E. G., Gomes, H., Gu, D., Guenther, A., Jathar, S. H., Kim, S., Liu, Y., Lou, S., Martin, S. T., McNeill, V. F., Medeiros, A., de Sá, S. S., Shilling, J. E., Springston, S. R., Souza, R. A. F., Thornton, J. A., Isaacman-VanWertz, G., Yee, L. D., Ynoue, R., Zaveri, R. A., Zelenyuk, A., and Zhao, C.: Urban pollution greatly enhances formation of natural aerosols over the Amazon rainforest, Nat. Commun., 10, 1046, https://doi.org/10.1038/s41467-019-08909-4, 2019.
Simon, M. C., Hudda, N., Naumova, E. N., Levy, J. I., Brugge, D., and Durant, J. L.: Comparisons of traffic-related ultrafine particle number concentrations measured in two urban areas by central, residential, and mobile monitoring, Atmo. Environ., 169, 113–127, https://doi.org/10.1016/j.atmosenv.2017.09.003, 2017.
Southerland, V. A., Brauer, M., Mohegh, A., Hammer, M. S., van Donkelaar, A., Martin, R. V., Apte, J. S., and Anenberg, S. C.: Global urban temporal trends in fine particulate matter (PM2.5) and attributable health burdens: estimates from global datasets, The Lancet Planet. Health, 6, e139–e146, https://doi.org/10.1016/S2542-5196(21)00350-8, 2022.
Stafoggia, M., Zauli-Sajani, S., Pey, J., Samoli, E., Alessandrini, E., Basagaña, X., Cernigliaro, A., Chiusolo, M., Demaria, M., Díaz, J., Faustini, A., Katsouyanni, K., Kelessis, A. G., Linares, C., Marchesi, S., Medina, S., Pandolfi, P., Pérez, N., Querol, X., Randi, G., Ranzi, A., Tobias, A., and Forastiere, F.: Desert Dust Outbreaks in Southern Europe: Contribution to Daily PM2.5 Concentrations and Short-Term Associations with Mortality and Hospital Admissions, Environ. Health Persp., 124, 413–419, https://doi.org/10.1289/ehp.1409164, 2016.
Stawovy, L. and Balakrishnan, B.: The Threat of Wildfires and Pulmonary Complications: A Narrative Review, Curr. Pulmonol. Rep., 11, 99–105, https://doi.org/10.1007/s13665-022-00293-7, 2022.
Stefenelli, G., Jiang, J., Bertrand, A., Bruns, E. A., Pieber, S. M., Baltensperger, U., Marchand, N., Aksoyoglu, S., Prévôt, A. S. H., Slowik, J. G., and El Haddad, I.: Secondary organic aerosol formation from smoldering and flaming combustion of biomass: a box model parametrization based on volatility basis set, Atmos. Chem. Phys., 19, 11461–11484, https://doi.org/10.5194/acp-19-11461-2019, 2019.
Stolk, R. P., Rosmalen, J. G. M., Postma, D. S., de Boer, R. A., Navis, G., Slaets, J. P. J., Ormel, J., and Wolffenbuttel, B. H. R.: Universal risk factors for multifactorial diseases, Eur. J. Epidemiol., 23, 67–74, https://doi.org/10.1007/s10654-007-9204-4, 2008.
Stone, R.: Counting the Cost of London's Killer Smog, Science, 298, 2106–2107, https://doi.org/10.1126/science.298.5601.2106b, 2002.
Strak, M., Weinmayr, G., Rodopoulou, S., Chen, J., de Hoogh, K., Andersen, Z. J., Atkinson, R., Bauwelinck, M., Bekkevold, T., Bellander, T., Boutron-Ruault, M.-C., Brandt, J., Cesaroni, G., Concin, H., Fecht, D., Forastiere, F., Gulliver, J., Hertel, O., Hoffmann, B., Hvidtfeldt, U. A., Janssen, N. A. H., Jöckel, K.-H., Jørgensen, J. T., Ketzel, M., Klompmaker, J. O., Lager, A., Leander, K., Liu, S., Ljungman, P., Magnusson, P. K. E., Mehta, A. J., Nagel, G., Oftedal, B., Pershagen, G., Peters, A., Raaschou-Nielsen, O., Renzi, M., Rizzuto, D., van der Schouw, Y. T., Schramm, S., Severi, G., Sigsgaard, T., Sørensen, M., Stafoggia, M., Tjønneland, A., Verschuren, W. M. M., Vienneau, D., Wolf, K., Katsouyanni, K., Brunekreef, B., Hoek, G., and Samoli, E.: Long term exposure to low level air pollution and mortality in eight European cohorts within the ELAPSE project: pooled analysis, BMJ, 374, n1904, https://doi.org/10.1136/bmj.n1904, 2021.
Strawa, A. W., Chatfield, R. B., Legg, M., Scarnato, B., and Esswein, R.: Improving retrievals of regional fine particulate matter concentrations from Moderate Resolution Imaging Spectroradiometer (MODIS) and Ozone Monitoring Instrument (OMI) multisatellite observations, J. Air Waste Manage. Assoc., 63, 1434–1446, https://doi.org/10.1080/10962247.2013.822838, 2013.
Su, H., Cheng, Y., and Pöschl, U.: New Multiphase Chemical Processes Influencing Atmospheric Aerosols, Air Quality, and Climate in the Anthropocene, Account. Chem. Res., 53, 2034–2043, https://doi.org/10.1021/acs.accounts.0c00246, 2020.
Sudlow, C., Gallacher, J., Allen, N., Beral, V., Burton, P., Danesh, J., Downey, P., Elliott, P., Green, J., Landray, M., Liu, B., Matthews, P., Ong, G., Pell, J., Silman, A., Young, A., Sprosen, T., Peakman, T., and Collins, R.: UK Biobank: An Open Access Resource for Identifying the Causes of a Wide Range of Complex Diseases of Middle and Old Age, PLOS Medicine, 12, e1001779, https://doi.org/10.1371/journal.pmed.1001779, 2015.
Suleiman, A., Tight, M. R., and Quinn, A. D.: Hybrid Neural Networks and Boosted Regression Tree Models for Predicting Roadside Particulate Matter, Environ. Model. Assess., 21, 731–750, https://doi.org/10.1007/s10666-016-9507-5, 2016.
Timmers, V. R. J. H. and Achten, P. A. J.: Non-exhaust PM emissions from electric vehicles, Atmos. Environ., 134, 10–17, https://doi.org/10.1016/j.atmosenv.2016.03.017, 2016.
Tong, Y., Pospisilova, V., Qi, L., Duan, J., Gu, Y., Kumar, V., Rai, P., Stefenelli, G., Wang, L., Wang, Y., Zhong, H., Baltensperger, U., Cao, J., Huang, R.-J., Prévôt, A. S. H., and Slowik, J. G.: Quantification of solid fuel combustion and aqueous chemistry contributions to secondary organic aerosol during wintertime haze events in Beijing, Atmos. Chem. Phys., 21, 9859–9886, https://doi.org/10.5194/acp-21-9859-2021, 2021.
Tsai, M. Y., Hoek, G., Eeftens, M., de Hoogh, K., Beelen, R., Beregszászi, T., Cesaroni, G., Cirach, M., Cyrys, J., De Nazelle, A., de Vocht, F., Ducret-Stich, R., Eriksen, K., Galassi, C., Gražuleviciene, R., Gražulevicius, T., Grivas, G., Gryparis, A., Heinrich, J., Hoffmann, B., Iakovides, M., Keuken, M., Krämer, U., Künzli, N., Lanki, T., Madsen, C., Meliefste, K., Merritt, A. S., Mölter, A., Mosler, G., Nieuwenhuijsen, M. J., Pershagen, G., Phuleria, H., Quass, U., Ranzi, A., Schaffner, E., Sokhi, R., Stempfelet, M., Stephanou, E., Sugiri, D., Taimisto, P., Tewis, M., Udvardy, O., Wang, M., and Brunekreef, B.: Spatial variation of PM elemental composition between and within 20 European study areas–Results of the ESCAPE project, Environ. Int., 84, 181–192, https://doi.org/10.1016/j.envint.2015.04.015, 2015.
Turner, M. C., Andersen, Z. J., Baccarelli, A., Diver, W. R., Gapstur, S. M., Pope III, C. A., Prada, D., Samet, J., Thurston, G., and Cohen, A.: Outdoor air pollution and cancer: An overview of the current evidence and public health recommendations, CA: A Cancer Journal for Clinicians, 70, 460–479, https://doi.org/10.3322/caac.21632, 2020.
van Donkelaar, A., Martin, R. V., Li, C., and Burnett, R. T.: Regional Estimates of Chemical Composition of Fine Particulate Matter Using a Combined Geoscience-Statistical Method with Information from Satellites, Models, and Monitors, Environ. Sci. Technol., 53, 2595–2611, https://doi.org/10.1021/acs.est.8b06392, 2019.
Van Ryswyk, K., Anastasopolos, A. T., Evans, G., Sun, L., Sabaliauskas, K., Kulka, R., Wallace, L., and Weichenthal, S.: Metro Commuter Exposures to Particulate Air Pollution and PM2.5-Associated Elements in Three Canadian Cities: The Urban Transportation Exposure Study, Environ. Sci. Technol., 51, 5713–5720, https://doi.org/10.1021/acs.est.6b05775, 2017.
Vasseur, E.: United Nations Conference on the Human Environment: Stockholm, 5–16 June 1972, Water Res., 7, 1227–1233, 1973.
Volz, A. and Kley, D.: Evaluation of the Montsouris series of ozone measurements made in the nineteenth century, Nature, 332, 240–242, https://doi.org/10.1038/332240a0, 1988.
Wang, H., Wang, H., Lu, X., Lu, K., Zhang, L., Tham, Y. J., Shi, Z., Aikin, K., Fan, S., Brown, S. S., and Zhang, Y.: Increased night-time oxidation over China despite widespread decrease across the globe, Nat. Geosci., 16, 217–223, https://doi.org/10.1038/s41561-022-01122-x, 2023a.
Wang, J., Li, J., Ye, J., Zhao, J., Wu, Y., Hu, J., Liu, D., Nie, D., Shen, F., Huang, X., Huang, D. D., Ji, D., Sun, X., Xu, W., Guo, J., Song, S., Qin, Y., Liu, P., Turner, J. R., Lee, H. C., Hwang, S., Liao, H., Martin, S. T., Zhang, Q., Chen, M., Sun, Y., Ge, X., and Jacob, D. J.: Fast sulfate formation from oxidation of SO2 by NO2 and HONO observed in Beijing haze, Nat. Commun., 11, 2844, https://doi.org/10.1038/s41467-020-16683-x, 2020.
Wang, M., Beelen, R., Basagana, X., Becker, T., Cesaroni, G., de Hoogh, K., Dedele, A., Declercq, C., Dimakopoulou, K., Eeftens, M., Forastiere, F., Galassi, C., Graþulevièienë, R., Hoffmann, B., Heinrich, J., Iakovides, M., Künzli, N., Korek, M., Lindley, S., Mölter, A., Mosler, G., Madsen, C., Nieuwenhuijsen, M., Phuleria, H., Pedeli, X., Raaschou-Nielsen, O., Ranzi, A., Stephanou, E., Sugiri, D., Stempfelet, M., Tsai, M.-Y., Lanki, T., Udvardy, O., Varró, M. J., Wolf, K., Weinmayr, G., Yli-Tuomi, T., Hoek, G., and Brunekreef, B.: Evaluation of Land Use Regression Models for NO2 and Particulate Matter in 20 European Study Areas: The ESCAPE Project, Environ. Sci. Technol., 47, 4357–4364, https://doi.org/10.1021/es305129t, 2013.
Wang, S., Gallimore, P. J., Liu-Kang, C., Yeung, K., Campbell, S. J., Utinger, B., Liu, T., Peng, H., Kalberer, M., Chan, A. W. H., and Abbatt, J. P. D.: Dynamic Wood Smoke Aerosol Toxicity during Oxidative Atmospheric Aging, Environ. Sci. Technol., 57, 1246–1256, https://doi.org/10.1021/acs.est.2c05929, 2023b.
Wang, Y., Shi, L., Lee, M., Liu, P., Di, Q., Zanobetti, A., and Schwartz, J. D.: Long-term Exposure to PM2.5 and Mortality Among Older Adults in the Southeastern US, Epidemiology, 28, 207–214 https://doi.org/10.1097/ede.0000000000000614, 2017.
Wang, Y., Xiao, S., Zhang, Y., Chang, H., Martin, R. V., Van Donkelaar, A., Gaskins, A., Liu, Y., Liu, P., and Shi, L.: Long-term exposure to PM2.5 major components and mortality in the southeastern United States, Environ. Int., 158, 106969, https://doi.org/10.1016/j.envint.2021.106969, 2022.
Weagle, C. L., Snider, G., Li, C., van Donkelaar, A., Philip, S., Bissonnette, P., Burke, J., Jackson, J., Latimer, R., Stone, E., Abboud, I., Akoshile, C., Anh, N. X., Brook, J. R., Cohen, A., Dong, J., Gibson, M. D., Griffith, D., He, K. B., Holben, B. N., Kahn, R., Keller, C. A., Kim, J. S., Lagrosas, N., Lestari, P., Khian, Y. L., Liu, Y., Marais, E. A., Martins, J. V., Misra, A., Muliane, U., Pratiwi, R., Quel, E. J., Salam, A., Segev, L., Tripathi, S. N., Wang, C., Zhang, Q., Brauer, M., Rudich, Y., and Martin, R. V.: Global Sources of Fine Particulate Matter: Interpretation of PM2.5 Chemical Composition Observed by SPARTAN using a Global Chemical Transport Model, Environ. Sci. Technol., 52, 11670–11681, https://doi.org/10.1021/acs.est.8b01658, 2018.
Weakley, A. T., Takahama, S., and Dillner, A. M.: Ambient aerosol composition by infrared spectroscopy and partial least-squares in the chemical speciation network: Organic carbon with functional group identification, Aerosol Sci. Technol., 50, 1096–1114, https://doi.org/10.1080/02786826.2016.1217389, 2016.
Wei, L., Kwan, M.-P., Vermeulen, R., and Helbich, M.: Measuring environmental exposures in people's activity space: The need to account for travel modes and exposure decay, J. Expo. Sci. Env. Epid., 33, 954–962, https://doi.org/10.1038/s41370-023-00527-z, 2023.
WHO: WHO Global Air Quality Guidelines, https://iris.who.int/bitstream/handle/10665/345334/9789240034433-eng.pdf (last access: 29 August 2024), 2021.
Wimo, A., Jönsson, L., Bond, J., Prince, M., Winblad, B., and Alzheimer Disease, I.: The worldwide economic impact of dementia 2010, Alzheimers Dement., 9, 1–11.e13, https://doi.org/10.1016/j.jalz.2012.11.006, 2013.
Wolf, K., Cyrys, J., Harciníková, T., Gu, J., Kusch, T., Hampel, R., Schneider, A., and Peters, A.: Land use regression modeling of ultrafine particles, ozone, nitrogen oxides and markers of particulate matter pollution in Augsburg, Germany, Sci. Total Environ., 579, 1531–1540, https://doi.org/10.1016/j.scitotenv.2016.11.160, 2017.
Wong, J. P. S., Yang, Y., Fang, T., Mulholland, J. A., Russell, A. G., Ebelt, S., Nenes, A., and Weber, R. J.: Fine Particle Iron in Soils and Road Dust Is Modulated by Coal-Fired Power Plant Sulfur, Environ. Sci. Technol., 54, 7088–7096, https://doi.org/10.1021/acs.est.0c00483, 2020.
Wyzga, R. E. and Rohr, A. C.: Long-term particulate matter exposure: Attributing health effects to individual PM components, J. Air Waste Manage. Assoc., 65, 523–543, https://doi.org/10.1080/10962247.2015.1020396, 2015.
Xu, L., Guo, H., Boyd, C. M., Klein, M., Bougiatioti, A., Cerully, K. M., Hite, J. R., Isaacman-VanWertz, G., Kreisberg, N. M., Knote, C., Olson, K., Koss, A., Goldstein, A. H., Hering, S. V., de Gouw, J., Baumann, K., Lee, S.-H., Nenes, A., Weber, R. J., and Ng, N. L.: Effects of anthropogenic emissions on aerosol formation from isoprene and monoterpenes in the southeastern United States, P. Natl. Acad. Sci. USA, 112, 37–42, https://doi.org/10.1073/pnas.1417609112, 2015.
Xu, L., Liu, X., Gao, H., Yao, X., Zhang, D., Bi, L., Liu, L., Zhang, J., Zhang, Y., Wang, Y., Yuan, Q., and Li, W.: Long-range transport of anthropogenic air pollutants into the marine air: insight into fine particle transport and chloride depletion on sea salts, Atmos. Chem. Phys., 21, 17715–17726, https://doi.org/10.5194/acp-21-17715-2021, 2021.
Xue, T., Zheng, Y., Tong, D., Zheng, B., Li, X., Zhu, T., and Zhang, Q.: Spatiotemporal continuous estimates of PM2.5 concentrations in China, 2000–2016: A machine learning method with inputs from satellites, chemical transport model, and ground observations, Environ. Int., 123, 345–357, https://doi.org/10.1016/j.envint.2018.11.075, 2019.
Yang, Y., Guo, Y., Qian, Z., Ruan, Z., Zheng, Y., Woodward, A., Ai, S., Howard, S. W., Vaughn, M. G., Ma, W., Wu, F., and Lin, H.: Ambient fine particulate pollution associated with diabetes mellitus among the elderly aged 50 years and older in China, Environ. Pollut., 243, 815–823, https://doi.org/10.1016/j.envpol.2018.09.056, 2018.
Yang, Y., Ruan, Z., Wang, X., Yang, Y., Mason, T. G., Lin, H., and Tian, L.: Short-term and long-term exposures to fine particulate matter constituents and health: A systematic review and meta-analysis, Environ. Pollut., 247, 874–882, https://doi.org/10.1016/j.envpol.2018.12.060, 2019.
Yao, L., Garmash, O., Bianchi, F., Zheng, J., Yan, C., Kontkanen, J., Junninen, H., Mazon, S. B., Ehn, M., Paasonen, P., Sipilä, M., Wang, M., Wang, X., Xiao, S., Chen, H., Lu, Y., Zhang, B., Wang, D., Fu, Q., Geng, F., Li, L., Wang, H., Qiao, L., Yang, X., Chen, J., Kerminen, V.-M., Petäjä, T., Worsnop, D. R., Kulmala, M., and Wang, L.: Atmospheric new particle formation from sulfuric acid and amines in a Chinese megacity, Science, 361, 278–281, https://doi.org/10.1126/science.aao4839, 2018.
Yazdani, A., Dudani, N., Takahama, S., Bertrand, A., Prévôt, A. S. H., El Haddad, I., and Dillner, A. M.: Characterization of primary and aged wood burning and coal combustion organic aerosols in an environmental chamber and its implications for atmospheric aerosols, Atmos. Chem. Phys., 21, 10273–10293, https://doi.org/10.5194/acp-21-10273-2021, 2021.
Yazdani, A., Dudani, N., Takahama, S., Bertrand, A., Prévôt, A. S. H., El Haddad, I., and Dillner, A. M.: Fragment ion–functional group relationships in organic aerosols using aerosol mass spectrometry and mid-infrared spectroscopy, Atmos. Meas. Tech., 15, 2857–2874, https://doi.org/10.5194/amt-15-2857-2022, 2022.
Yu, P., Xu, R., Li, S., Yue, X., Chen, G., Ye, T., Coêlho, M. S. Z. S., Saldiva, P. H. N., Sim, M. R., Abramson, M. J., and Guo, Y.: Exposure to wildfire-related PM2.5 and site-specific cancer mortality in Brazil from 2010 to 2016: A retrospective study, PLOS Medicine, 19, e1004103, https://doi.org/10.1371/journal.pmed.1004103, 2022.
Yuchi, W., Sbihi, H., Davies, H., Tamburic, L., and Brauer, M.: Road proximity, air pollution, noise, green space and neurologic disease incidence: a population-based cohort study, Environ. Health, 19, 8, https://doi.org/10.1186/s12940-020-0565-4, 2020.
Zeger, S. L., Thomas, D., Dominici, F., Samet, J. M., Schwartz, J., Dockery, D., and Cohen, A.: Exposure measurement error in time-series studies of air pollution: concepts and consequences, Environ. Health Persp., 108, 419–426, https://doi.org/10.1289/ehp.00108419, 2000.
Zhan, Y., Luo, Y., Deng, X., Chen, H., Grieneisen, M. L., Shen, X., Zhu, L., and Zhang, M.: Spatiotemporal prediction of continuous daily PM2.5 concentrations across China using a spatially explicit machine learning algorithm, Atmos. Environ., 155, 129–139, https://doi.org/10.1016/j.atmosenv.2017.02.023, 2017.
Zhang, H., Shi, L., Ebelt, S. T., D'Souza, R. R., Schwartz, J. D., Scovronick, N., and Chang, H. H.: Short-term associations between ambient air pollution and emergency department visits for Alzheimer's disease and related dementias, Environ. Epidemiol., 7, e237, https://doi.org/10.1097/ee9.0000000000000237, 2023.
Zhang, J. J. Y., Sun, L., Barrett, O., Bertazzon, S., Underwood, F. E., and Johnson, M.: Development of land-use regression models for metals associated with airborne particulate matter in a North American city, Atmos. Environ., 106, 165–177, https://doi.org/10.1016/j.atmosenv.2015.01.008, 2015.
Zhang, X., Zhao, L., Tong, D. Q., Wu, G., Dan, M., and Teng, B.: A Systematic Review of Global Desert Dust and Associated Human Health Effects, Atmosphere, 7, 158, https://doi.org/10.3390/atmos7120158, 2016.
Zhou, S., Collier, S., Jaffe, D. A., Briggs, N. L., Hee, J., Sedlacek III, A. J., Kleinman, L., Onasch, T. B., and Zhang, Q.: Regional influence of wildfires on aerosol chemistry in the western US and insights into atmospheric aging of biomass burning organic aerosol, Atmos. Chem. Phys., 17, 2477–2493, https://doi.org/10.5194/acp-17-2477-2017, 2017.
Zins, M., Bonenfant, S., Carton, M., Coeuret-Pellicer, M., Guéguen, A., Gourmelen, J., Nachtigal, M., Ozguler, A., Quesnot, A., Ribet, C., Rodrigues, G., Serrano, A., Sitta, R., Brigand, A., Henny, J., and Goldberg, M.: The CONSTANCES cohort: an open epidemiological laboratory, BMC Public Health, 10, 479, https://doi.org/10.1186/1471-2458-10-479, 2010.
PM2.5 and PM10: particulate matter with a size lower than 2.5 and 10 µm, respectively.
The Surface Particulate Matter Network (SPARTAN) provides publicly available data on PM mass, chemical composition, and optical characteristics for integration with satellite remote sensing and for air quality management.
The European Monitoring and Evaluation Programme (EMEP) aims to monitor and model the long-range transport of air pollutants across Europe.
The Interagency Monitoring of Protected Visual Environments (IMPROVE) network is a long-term monitoring programme designed to assess visibility and air quality in national parks and wilderness areas in the United States. The primary goal of the IMPROVE network is to measure PM mass and chemical composition at over 170 monitoring sites across the United States.
The Aerosol, Clouds and Trace Gases Research Infrastructure (ACTRIS) is a pan-European research infrastructure consisting of several measurement stations across Europe, providing long-term observational data on aerosols, clouds, and trace gases.
The Atmospheric Science and Chemistry mEasurement NeTwork (ASCENT) is a new, comprehensive, high-time-resolution, long-term measurement network in the US intended for the characterization of aerosol chemical composition and physical properties.
- Abstract
- Preamble
- Integrating PM chemical composition into health studies
- Modelling exposures to individual PM components
- Field observations required for understanding PM differential toxicity
- Gaps in understanding emissions
- Supporting epidemiology by enhancing chemically resolved PM exposure estimation
- Conclusions
- Code availability
- Data availability
- Author contributions
- Competing interests
- Disclaimer
- Special issue statement
- Acknowledgements
- Financial support
- Review statement
- References
- Abstract
- Preamble
- Integrating PM chemical composition into health studies
- Modelling exposures to individual PM components
- Field observations required for understanding PM differential toxicity
- Gaps in understanding emissions
- Supporting epidemiology by enhancing chemically resolved PM exposure estimation
- Conclusions
- Code availability
- Data availability
- Author contributions
- Competing interests
- Disclaimer
- Special issue statement
- Acknowledgements
- Financial support
- Review statement
- References