the Creative Commons Attribution 4.0 License.
the Creative Commons Attribution 4.0 License.
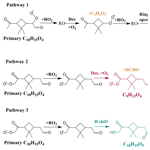
Direct probing of acylperoxy radicals during ozonolysis of α-pinene: constraints on radical chemistry and production of highly oxygenated organic molecules
Han Zang
Dandan Huang
Jiali Zhong
Chenxi Li
Huayun Xiao
Acylperoxy radicals (RO2) are key intermediates in the atmospheric oxidation of organic compounds and different from the general alkyl RO2 radicals in reactivity. However, direct probing of the molecular identities and chemistry of acyl RO2 remains quite limited. Here, we report a combined experimental and kinetic modeling study of the composition and formation mechanisms of acyl RO2, as well as their contributions to the formation of highly oxygenated organic molecules (HOMs) during ozonolysis of α-pinene. We find that acyl RO2 radicals account for 67 %, 94 %, and 32 % of the highly oxygenated C7, C8, and C9 RO2, respectively, but only a few percent of C10 RO2. The formation pathway of acyl RO2 species depends on their oxygenation level. The highly oxygenated acyl RO2 (oxygen atom number ≥6) are mainly formed by the intramolecular aldehydic H shift (i.e., autoxidation) of RO2, while the less oxygenated acyl RO2 (oxygen atom number <6) are basically derived from the C–C bond cleavage of alkoxy (RO) radicals containing an α-ketone group or the intramolecular H shift of RO containing an aldehyde group. The acyl-RO2-involved reactions explain 50 %–90 % of C7 and C8 closed-shell HOMs and 14 % of C10 HOMs, respectively. For C9 HOMs, this contribution can be up to 30 %–60 %. In addition, acyl RO2 contribute to 50 %–95 % of C14–C18 HOM dimer formation. Because of the generally fast reaction kinetics of acyl RO2, the acyl RO2 + alkyl RO2 reactions seem to outcompete the alkyl RO2 + alkyl RO2 pathways, thereby affecting the fate of alkyl RO2 and HOM formation. Our study sheds lights on the detailed formation pathways of the monoterpene-derived acyl RO2 and their contributions to HOM formation, which will help to understand the oxidation chemistry of monoterpenes and sources of low-volatility organic compounds capable of driving particle formation and growth in the atmosphere.
- Article
(5912 KB) - Full-text XML
-
Supplement
(2055 KB) - BibTeX
- EndNote
Monoterpenes (C10H16) comprise an important fraction of nonmethane hydrocarbons in the global atmosphere (Guenther et al., 2012; Sindelarova et al., 2014) and make a significant contribution to the secondary organic aerosol (SOA) budget (Pye et al., 2010; Iyer et al., 2021). The presence of a double bond and the large molecular size of monoterpenes favor their oxidation reactivity towards O3, hydroxyl (OH), and nitrate (NO3) radicals (Atkinson et al., 1990, 1986; Kurten et al., 2015; Kristensen et al., 2016; Bianchi et al., 2019; Berndt, 2022), as well as the formation of low-volatility products and SOA (Fry et al., 2009, 2014; Zhang et al., 2018; Bianchi et al., 2019; Molteni et al., 2019; Shen et al., 2022). The organic peroxy radicals (RO2) in the gas-phase oxidation of monoterpenes can undergo autoxidation and form a class of highly oxygenated organic molecules (HOMs) (Jokinen et al., 2014; Mentel et al., 2015; Berndt et al., 2016; Zhao et al., 2018; Bianchi et al., 2019; Bell et al., 2022; Berndt, 2022), which are primarily low-volatility or extremely low-volatility organic compounds (LVOCs and ELVOCs) (Ehn et al., 2014; Bianchi et al., 2019) and thus play a crucial role in SOA formation and growth.
Significant advances have been made in recent years concerning the monoterpene RO2 autoxidation and its contribution to HOM formation (Ehn et al., 2014; Berndt et al., 2016; Zhao et al., 2018; Xu et al., 2019; Lin et al., 2021; Berndt, 2022; Shen et al., 2022). It is recognized that some monoterpene RO2 radicals derived from the traditional ozonolysis channel (i.e., isomerization of Criegee intermediates, CIs) and OH addition channel can autoxidize at a rate larger than 1 s−1 and could be an important contributor to HOM formation (Zhao et al., 2018; Xu et al., 2019; Berndt, 2021). Recently, new reaction channels leading to the RO2 radicals that can undergo fast autoxidation have been proposed. A quantum chemical calculation study indicated that an excited CI arising from α-pinene ozonolysis could undergo ring-breaking reactions and directly lead to a ring-opened RO2 due to the excess energy, which can autoxidize at a rate of ∼1 s−1 and rapidly form highly oxidized RO2 with up to 8 oxygen atoms (Iyer et al., 2021). In addition, the minor hydrogen abstraction channel by OH radicals has been proposed as a predominant pathway to HOM formation from OH oxidation of α-pinene under atmospheric conditions (Shen et al., 2022).
RO2 species can be simply divided into alkyl RO2 and acyl RO2 (RC(O)OO) according to whether R is an acyl radical. There are significant differences in the reactivity of these two kinds of RO2. Firstly, the rate constant of acyl RO2 with NO is in general slightly higher than that of alkyl RO2 (Atkinson et al., 2007; Calvert et al., 2008; Orlando and Tyndall, 2012). For example, the reaction rate constants of acyl RO2, CH3C(O)O2, and alkyl RO2, CH3CH2O2, with NO have been reported to be and , respectively (Atkinson et al., 2007; Calvert et al., 2008; Orlando and Tyndall, 2012). Besides, acyl RO2 can react rapidly with NO2 and form thermally unstable peroxyacyl nitrates (RC(O)OONO2), which have a lifetime of tens of minutes at room temperature and of days and even months in winter or in the upper atmosphere with lower temperatures (Atkinson et al., 2007; Orlando and Tyndall, 2012). Although alkyl RO2 radicals can also react with NO2 and form the alkyl peroxynitrates (ROONO2), they are extremely unstable and will decompose into RO2 radicals and NO2 in less than 1 s (Kirchner et al., 1997; Orlando and Tyndall, 2012). Lastly, the rate constant of cross-reaction of acyl RO2 ( ) is significantly higher than that of alkyl RO2 (– ) (Villenave and Lesclaux, 1996; Tyndall et al., 2001; Atkinson et al., 2007; Zhao et al., 2018). As a result, these two kinds of RO2 may play different roles in the autoxidation as well as HOM and dimer formation.
The quantum calculations revealed that different functional groups in RO2 would lead to significantly different intramolecular H-shift rates (Otkjær et al., 2018). The C=O and C=C substituents led to resonance-stabilized carbon radicals and could enhance the H-shift rate constants by more than a factor of 400. The fast aldehydic H-shift rate contributes to a series of acyl radicals (RC(O)) with the radical site at the terminal carbonyl carbon, which further produce the acyl RO2 with O2 addition. Many RO2 formed in the oxidation of monoterpenes have the aldehyde functionality, especially for α-pinene ozonolysis, in which all the primary and many later-generation RO2 contain at least one aldehyde group (Noziere et al., 2015; Berndt et al., 2018; Li et al., 2019; Berndt, 2022; Zhao et al., 2022). As a result, acyl RO2 may comprise a considerable fraction of total RO2 species and contribute significantly to the formation of low-volatility products and SOA in the monoterpene oxidation system. A recent study by Zhao et al. (2022) found that the acyl-RO2-involved reactions contribute to 50 %–80 % of oxygenated C15–C20 dimers () and 70 % of C15–C19 dimer esters in SOA from α-pinene ozonolysis. However, currently the direct probing of the molecular identities and chemistry of monoterpene-derived acyl RO2 radicals is rather limited. The role of acyl RO2 in HOM formation remains to be quantified.
In this study, the molecular identities and formation mechanisms of acyl RO2 radicals, as well as their contributions to HOM formation in the α-pinene ozonolysis, are investigated. The experiments were conducted in a flow reactor with different concentrations of NO2, which acted as an efficient scavenger for the acyl RO2. The molecular composition and abundance of the gas-phase HOMs were measured by a chemical ionization–atmospheric pressure interface–time-of-flight mass spectrometer (CI-APi-ToF) using nitrate as the reagent ions. In addition, kinetic modeling using the Framework for 0-D Atmospheric Modeling (F0AM v4.1) employing the Master Chemical Mechanism (MCM v3.3.1), updated with the latest advances of the RO2 chemistry, was performed to gain insights into the reaction kinetics and mechanisms of acyl RO2 species. We find that acyl RO2 account for a major fraction of highly oxygenated C7 and C8 RO2 and play a significant role in the formation of HOM monomers and dimers with small molecular size. This study will help to understand the role of acyl RO2 in the formation of low-volatility species from monoterpene oxidation and reduce the uncertainties in the future atmospheric modeling of the formation and impacts of aerosols.
2.1 Flow reactor experiments
The α-pinene ozonolysis experiments were carried out under room temperature (298 K) and dry conditions (relative humidity <5 %) in a custom-built flow reactor, which has been described in detail previously (Yao et al., 2019). The α-pinene vapor was generated by evaporating its pure liquid (99 %, Sigma-Aldrich) into a flow of zero air (10.65 L min−1) added to the reactor using an automated syringe pump (TYD01–01 CE, Baoding Leifu Fluid Technology Co., Ltd.). The initial concentrations of α-pinene ranged from 500 ppb to 3 ppm in different experiments. Ozone was generated by passing a flow of ultra-high-purity (UHP) O2 (150 mL min−1, Shanghai Maytor Special Gas Co., Ltd.) through a quartz tube housing a pen-ray mercury lamp (UV-S2, UVP Inc.), and its concentration (45 and 180 ppb under low and high O3 conditions, respectively) was measured by an ozone analyzer (Model 49i, Thermo Fisher Scientific, USA). The NO2, acting as an acyl RO2 scavenger, was derived from its standard cylinder gas (15.6 ppm, Shanghai Weichuang Standard Gas Co., Ltd.), and its initial concentration ranged from 0 to 30 ppb. To validate the formation mechanisms of acyl RO2, selected experiments with the addition of NO or cyclohexane were also conducted. NO was derived by its standard cylinder gas (9.8 ppm, Shanghai Weichuang Standard Gas Co., Ltd.), and its initial concentration also ranged from 0 to 30 ppb. The gas-phase cyclohexane (∼500 ppm), acting as an OH scavenger, was generated by bubbling a gentle flow of UHP N2 through liquid cyclohexane (LC-MS grade, CNW). The total air flow in the flow reactor was 10.8 L min−1, and the residence time was 25 s. The relatively low O3 concentration and short reaction time in the flow reactor avoid significant production of NO3 radicals from NO2 and O3 and make the NO3 oxidation contribute only 0.3 %–1.2 % of the total α-pinene oxidation in our experiments. Therefore, the NO3 chemistry could be neglected in this study. A summary of the experimental conditions is given in Tables S1 and S2 in the Supplement.
The gas-phase RO2 radicals and closed-shell products were measured by a nitrate-based CI-APi-ToF mass spectrometer (abbreviated as nitrate-CIMS; Aerodyne Research, Inc.), and a long time-of-flight mass spectrometer with a mass resolution of ∼10 000 was used here. The mass calibration error is below 1.8 ppm. The sheath flow, including a 2 mL min−1 UHP N2 flow containing nitric acid (HNO3) and 22.4 L min−1 zero air, was guided through the PhotoIonizer (Model L9491, Hamamatsu, Japan) to generate nitrate reagent ions. The total sample flow rate was 9 L min−1 during the experiments. The mass spectra within the range of 50 to 700 were analyzed using the tofTools package developed by Junninen et al. (2010) based on MATLAB.
To clarify whether there is SOA formation in the experiments, a scanning mobility particle sizer (SMPS, TSI), which consists of an electrostatic classifier (model 3082), a long or nano differential mobility analyzer (model 3081 and 3085 for different particle sizes), and a condensation particle counter (model 3756), was used to monitor the formation of SOA particles. Except in Experiment (Exp) 31 where the reacted α-pinene reached 36.8 ppb and there was low SOA formation with particle mass concentrations of – µg m−3 and number concentrations of 63–395 cm−3, no particle formation was observed by SMPS. Therefore, the RO2 radicals and closed-shell products would be primarily distributed in the gas phase, with their fates negligibly influenced by the low SOA formation under these experimental conditions.
2.2 Kinetic model simulations
Model simulations of RO2 and HOM formation in selected experiments were performed to constrain the reaction kinetics and mechanisms of acyl RO2 using F0AM v4.1 (Wolfe et al., 2016), which employs MCM v3.3.1 (Jenkin et al., 2015) updated with the chemistry of RO2 autoxidation and cross-reactions forming HOM monomers and dimers. Newly added species and reactions to MCM v3.3.1 followed the work by Zhao et al. (2018) and Wang et al. (2021). Considering that the default MCM v3.3.1 does not include highly oxygenated acyl RO2, we added the possible formation pathways of the potential acyl RO2 measured in this study to the model based on the mechanisms proposed by Zhao et al. (2022).
The formation and reaction branching ratios of the two α-pinene-derived CIs are updated in the model according to the recent studies (Table S3) (Claflin et al., 2018; Iyer et al., 2021; Zhao et al., 2021; Berndt, 2022). The formation of a ring-opened C10H15O4-RO2 species (C10H15O4RBRO2 in Table S3) from α-pinene ozonolysis proposed by a recent study (Iyer et al., 2021), as well as its subsequent autoxidation and bimolecular reactions, is included in the model. The autoxidation rate constant of the ring-opened C10H15O4-RO2 is 1 s−1, and a lower limit of its molar yield (30 %) was used according to the recent studies (Zhao et al., 2021; Meder et al., 2023) and our results (see details in Sect. 3.3). We also added the hydrogen abstraction channel of α-pinene oxidation by OH radicals according to a recent study (Shen et al., 2022). The branching ratio of this channel was set to 9 %, with the remaining 91 % being the traditional OH addition pathways. The detailed reaction pathways and rate constants of RO2 species in this channel followed the work by Shen et al. (2022), except for RO2 cross-reactions, the rates of which were not reported in that study. As the primary RO2 radicals (C10H15O2-RO2) formed via the hydrogen abstraction by OH radicals are least oxidized with only 2 oxygen atoms, their cross-reaction rate could be relatively low (Atkinson et al., 2007; Orlando and Tyndall, 2012). In the model, this rate constant was set to . For other alkyl RO2 radicals (including HOM-RO2), their cross-reaction rate constant is assumed to be according to Zhao et al. (2018). The dimer formation rates for these alkyl RO2 are same as their cross-reaction rates.
In flow reactor experiments, the equilibrium formation of ROONO2 would lead to the consumption of alkyl RO2 radicals. To account for the influence of this process on the RO2 budget and HOM formation, we included the reaction of in the model, with forward and reverse reaction rate constants of and 5 s−1, respectively (Orlando and Tyndall, 2012). To simplify the parameterization, the forward and reverse reaction rate constants of newly added highly oxygenated acyl RO2 with NO2 are the same as default values in MCM v3.3.1. Besides, the cross-reaction rate constants of acyl RO2 (including acyl RO2 + acyl RO2 and acyl RO2 + alkyl RO2) forming monomers or dimers were both set to (Orlando and Tyndall, 2012). Considering that there are large uncertainties in the dimer formation rate of RO2, a sensitivity analysis was conducted to evaluate its influence on acyl-RO2-involved HOM formation by varying the rate constant from to for alkyl RO2 and to for acyl RO2. The results show that changes in dimer formation rate constants within the above ranges have no significant influence on the contribution of acyl RO2 to HOM formation (Fig. S1 in the Supplement).
The wall losses of OH, HO2, and RO2 radicals, as well as closed-shell HOM monomers and dimers in the flow reactor, were considered using the Knopf–Pöschl–Shiraiwa (KPS) method proposed by Knopf et al. (2015) in the model (Table S4), with an assumption of irreversible uptake of these species on the reactor wall. It is found that the wall loss of OH, HO2, and RO2 radicals accounts for 0.08 %–0.14 %, 4.7 %–9.1 %, and 7.3 %–25.5 % of their total production, respectively, with lower values under higher reacted α-pinene concentration conditions. Therefore, the wall loss process would not significantly influence α-pinene oxidation and RO2 chemistry. The wall losses of closed-shell HOM monomers and dimers account for 18.4 %–34.7 % and 14.2 %–33.1 % of their total production, respectively. It should be noted that the wall losses of typical RO2 and HOMs have a negligible impact on their responses to the addition of NO2 (Fig. S2). In addition, with the consideration of the wall loss effects, the contribution of acyl RO2 to the HOM formation only changed a little (0.02 %–0.5 %). Therefore, the wall losses of RO2 and HOMs in the flow reactor would not affect the interpretation of the results in this study.
3.1 Molecular composition of acyl RO2 from α-pinene ozonolysis
The overall formation characteristics of gas-phase RO2, closed-shell monomers, and dimers with the addition of NO2 (30 ppb) are shown in Fig. 1 (Exps 8 and 14, Table S1). Since the nitrate-CIMS is only highly sensitive to the highly oxygenated species, we only discuss the production of HOMs with oxygen atoms above 6 here. As for RO2 and closed-shell monomers (Fig. 1a), the signals of C7 and C8 species decrease by more than 50 % with the addition of NO2, while for C9 and C10 species, their decreases are relatively small (within 40 %). In addition, we note that there is an unexpected increase in some C9 and C10 RO2, and the possible reason will be discussed in detail later.
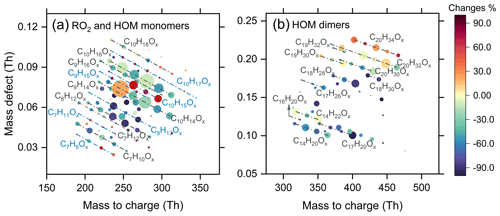
Figure 1Mass defect plots of (a) RO2 and HOM monomers and (b) HOM dimers formed from ozonolysis of α-pinene in the presence of NO2 measured using a nitrate-CIMS (Exps 8, 14). The circles are colored by the relative changes in signal of RO2, monomers, and dimers due to the addition of NO2 (30 ppb). The area of circles is linearly scaled with the cube root of the signal of HOMs formed in the absence of NO2. The blue lines represent RO2 radicals.
NO2 could react rapidly with acyl RO2 radicals to form RC(O)OONO2, which has a higher thermal-stability compared to ROONO2 and can serve as a sink for acyl RO2 on our experimental timescales. Therefore, a significant decrease in C7 and C8 RO2 and HOMs upon the addition of NO2 indicates that a major fraction of C7 and C8 RO2 are acyl RO2. In contrast, the slight decrease in C9 and C10 HOM monomers shows that the contribution of acyl RO2 to C9 and C10 RO2 is relatively small. However, some of the C10 monomers showed a slight increase with the addition of NO2, especially for C10H18Ox HOMs. The addition of NO2 plays a twofold role in dimer formation from α-pinene ozonolysis (Fig. 1b). There is a significant inhibiting effect on C14–C18 dimers, which is due to the large contribution of acyl RO2 to the total C7 and C8 RO2 that generate such dimers. However, C19 and C20 dimers only show a slight decrease with the addition of NO2, and some of them are even enhanced. In particular, the enhancement in C20H34Ox is most significant, reaching 30 %.
Kinetic model simulations show that the concentration of alkyl RO2 decreases by 1 %–20 % with the addition of 30 ppb NO2 under different reacted α-pinene conditions (Exps 1–28). Considering that the acyl RO2 could be rapidly consumed by NO2, if the signal reduction of a RO2 specie significantly exceeds 20 % with 30 ppb NO2 addition, we presume it has significant contribution from acyl RO2. As a result, a total of 10 acyl RO2 were identified according to the changes of RO2 signal as a function of initial NO2 concentration, which include C7H9O6, C7H9O7, C8H13O6, C8H13O8, C8H13O9, C8H13O10, C9H13O9, C9H17O7, C9H17O9, and C10H15O7. Figure 2 shows the averaged normalized acyl RO2 signals measured as a function of the added NO2 concentration under different experimental conditions (Exps 1–28). Similarly, since the nitrate-CIMS is only highly sensitive to products with high oxygen content, we only observed acyl RO2 with oxygen atoms above 6. Consistent with the significant decrease in C7 and C8 species with the addition of NO2 in Fig. 1a, C7 and C8 acyl RO2 decrease by more than 50 % with the increase in NO2 concentration (Fig. 2a and b). For C9 acyl RO2, the C9H17O7-RO2 and C9H17O9-RO2 also decrease dramatically with increasing NO2, and the decrease in C9H13O9-RO2 is relatively smaller (Fig. 2c). In addition, C10H15O7-RO2 also shows a small decrease (Fig. 2d), with a reduction of only 30 % at 30 ppb NO2. The relatively small reduction in the abundance of some of these RO2 radicals indicates the presence of alkyl RO2 radicals with the same chemical formulas. Along with the marked reduction in acyl RO2 signals, the production of highly oxygenated RC(O)OONO2 species such as C9H13O9NO2, C9H17O7NO2, and C10H15O7NO2 with the addition of NO2 was observed (see the spectra in Fig. S3). However, we note that although some RC(O)OONO2 such as C8H13O6NO2 and C8H13O8NO2 are expected to be formed with NO2 addition, they could not be unambiguously detected by the nitrate-CIMS due to the overlapping of their peaks with strong alkyl RO2 peaks (C9H15O8-RO2 and C9H15O10-RO2) in this study.
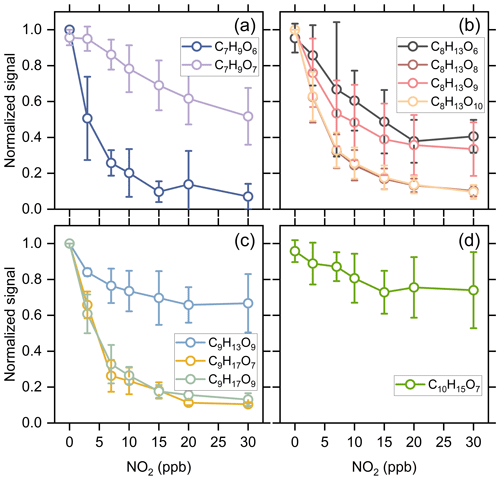
Figure 2Averaged normalized signal of the measured acyl RO2 as a function of the added NO2 concentration under different experimental conditions (Exps 1–28).
Figure 3 shows the contribution of acyl and alkyl RO2 to the highly oxygenated C7–C10 RO2. Acyl RO2 contribute 66.9 %, 94.3 % and 31.7 % to the total C7, C8, and C9 RO2 signals, respectively. By contrast, the only C10 acyl RO2 measured in this study is C10H15O7, which contributes to only 0.4 % of the total C10 RO2. It should be noted that there might be other C10 acyl RO2 that were not observed due to the interferences from the alkyl RO2 with the same chemical formulas, which respond differently to the addition of NO2 than acyl RO2 do (see details in the following discussion). Considering that some RO2 formulas such as C10H15O7 may have contributions from both acyl RO2 and alkyl RO2, we assumed the decrease of RO2 signal with the addition of NO2 as the signal of acyl RO2. Besides, it is obvious that the normalized signal basically decreases to the lowest value when the initial NO2 concentration reaches 10 ppb (Fig. 2), indicating that most of the acyl RO2 are depleted at this NO2 concentration. In addition, the decreasing extents of some acyl RO2 are different for different reacted α-pinene concentrations, with lower decreasing extent for higher reacted α-pinene concentrations (Fig. S4). This difference might be due to the promoted cross-reactions of acyl RO2 as well as their precursor RO2 at higher α-pinene concentrations, which are competitive with the reactions leading to acyl RO2 formation as well as the acyl RO2 + NO2 reactions.
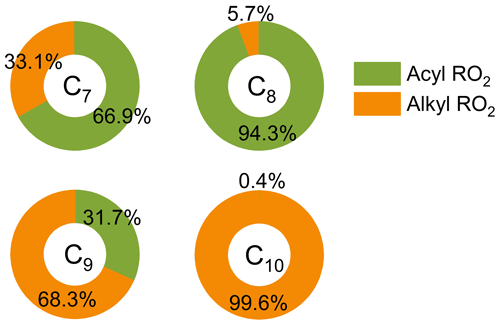
Figure 3Contributions of acyl and alkyl RO2 to the highly oxygenated C7–C10 RO2 measured by a nitrate-CIMS.
In addition to the changes of acyl RO2 signal, we also show the changes of normalized alkyl RO2 signal with the increasing initial NO2 concentration in Fig. S5. Although ROONO2 formed by the reaction of alkyl RO2 with NO2 is thermally unstable and would decompose quickly to release RO2, it would still reach a formation–decomposition equilibrium in the system, thus consuming a small amount of alkyl RO2. However, it can be seen from Fig. S5 that during 25 s of reaction in the flow reactor, a large part of alkyl RO2 has an increasing trend with the increase in NO2 concentration. We speculate that a portion of ROONO2 could decompose back to RO2 and NO2 in the nitrate chemical ionization inlet where the sample gases were diluted instantly and the equilibrium of ROONO2 was disturbed, resulting in the release of a large amount of RO2.
To verify our speculation, the decomposition of ROONO2 in the chemical ionization inlet was simulated based on the dilution ratio (1:3.5) and residence time (200 ms) in the inlet. As shown in Fig. S6, more than 40 % of ROONO2 decomposes back to RO2 and NO2 in the chemical ionization inlet, which would inevitably lead to an increase in RO2 concentration. As the C10H15O8NO2 has a significant contribution from the relatively stable RC(O)OONO2 arising from the ring-opened acyl C10H15O8-RO2 reported by Iyer et al. (2021), its decomposition is relatively small (∼21 %). It should be noted that the RO2 measured here is only a part of total RO2 produced and that a large amount of RO2 has already reacted to form closed-shell products as well as ROONO2 in the flow reactor. Taking Exp 14 as an example (30 ppb NO2), the simulated concentrations of RO2 and ROONO2 are 1.3 and 1.9 ppb, which approximately accounts for 27.1 % and 39.6 % of the total production of RO2, respectively. Therefore, the decomposition of ROONO2 could indeed result in an increase in the RO2 signal. It should also be pointed out that because of the very short residence time in the chemical ionization inlet, such an increase in the RO2 concentration would not significantly impact HOM formation.
To confirm the reliability of our results, we examined the changes in the signals of RO2 and closed-shell products as a function of reacted α-pinene in the absence of NO2 (Sect. S1 and Fig. S7 in the Supplement), and the results are consistent with previous studies (Zhao et al., 2018). In addition, we repeated Exps 15–21 on another nitrate-CIMS, and a similar increase in alkyl RO2 signals with the addition of NO2 was observed on that instrument (Fig. S8).
3.2 Formation mechanisms of acyl RO2 during α-pinene ozonolysis
It has been recently suggested that there are three main pathways that directly lead to the formation of monoterpene-derived acyl RO2 (Zhao et al., 2022): (i) the autoxidation of RO2 containing aldehyde groups (Reaction R1), (ii) the cleavage of a C–C bond of RO containing an α-ketone group (Reaction R2), and (iii) the intramolecular H shift of RO containing an aldehyde group (Reaction R3). In addition, the secondary OH oxidation of aldehyde products can also produce acyl RO2 radicals. However, in the present study, the secondary OH oxidation is expected to be insignificant due to an excess of α-pinene compared to O3. Indeed, kinetic model simulations incorporating the secondary OH chemistry show that the contribution of secondary OH oxidation to acyl RO2 formation is negligible even under high O3 conditions (see details in Sect. S2 and Fig. S9).
Here, we further investigated the formation mechanisms of acyl RO2. Figure 4 shows the reaction schemes leading to the formation of example acyl RO2 radicals. The detailed formation mechanisms of acyl RO2 measured in this study are shown in Fig. S10. The formation of acyl RO2, especially those having the small molecular size (C7–C9), requires the production and subsequent decomposition (or ring-opening process) of RO radicals. Taking C8H13O6-RO2 as an example (Fig. 4), two steps of RO formation and decomposition following the primary C10H15O4-RO2 lead to the ring-opened C8H13O4-RO2 that can undergo rapid aldehydic H shift to form the acyl RO2.
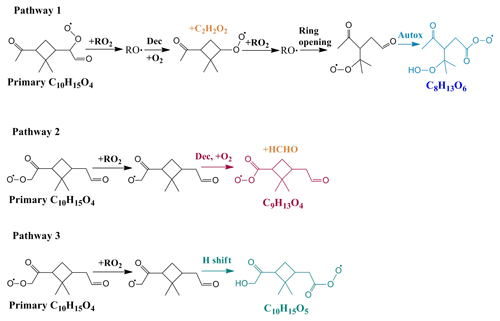
Figure 4Three different formation pathways of acyl RO2 during ozonolysis of α-pinene. The acyl RO2, C9H13O4 and C10H15O5, formed via pathways 2 and 3, respectively, were not detected by the nitrate-CIMS in this study due to their relatively low oxygenation level.
To verify the formation mechanisms of acyl RO2, we added NO in some experiments (Exps 33–56) to see how acyl RO2 respond to the increasing NO concentration. As shown in Fig. 5, the changes of C7 and C8 acyl RO2 show an opposite trend with the increasing NO and NO2 concentration, except for C8H13O8-RO2. NO can react with RO2 to form RO radicals and promote the formation of RO2 that requires the involvement of RO radicals in their formation. In addition to C8H13O6-RO2 discussed above, the formation of C7H9O7-RO2 and C8H13O9-RO2 needs two and four steps of the RO formation following C10H15O4-RO2 (Fig. S10), respectively. Therefore, the increase in RO concentration due to the addition of NO would promote the production of these acyl RO2. These results prove that the RO radicals indeed play an important role in the acyl RO2 formation, while for C8H13O8-RO2, its signal decreases substantially with the addition of NO up to 3 ppb, similar to the trend observed with the addition of NO2. After reaching the minimum at 7 ppb NO, the signal of C8H13O8-RO2 tends to increase with the further increase in NO concentration. Given that C8H13O8-RO2 is likely to directly come from the autoxidation of C8H13O6 acyl RO2 (see Fig. S10), the rapid consumption of C8H13O6-RO2 by NO and NO2 (formed by O3 oxidation of NO) may outcompete its autoxidation process, thus leading to a decrease in C8H13O8-RO2 signal. Besides, it can be seen that the increasing extent in C8H13O6-RO2 is also relatively small before the NO concentration reaches 3 ppb (Fig. 5c), indicating that the promotion effect of NO on C8H13O6-RO2 formation is not that strong at this concentration.
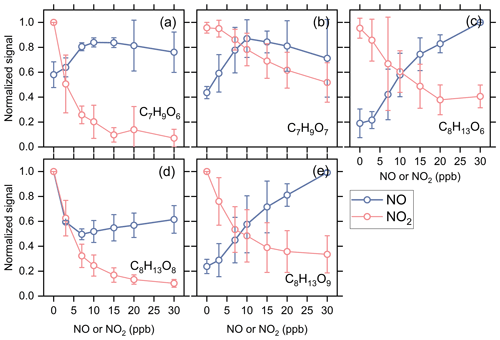
Figure 5Averaged normalized signal of typical acyl RO2 as a function of initial NO or NO2 addition (Exps 1–28 and 33–56).
It is interesting to note that most of the measured highly oxygenated acyl RO2 are formed by the autoxidation of aldehydic RO2, and only the C8H13O9-RO2 is formed by the H shift of the RO radical (Fig. S10). The measured signal of acyl RO2 from the autoxidation pathway accounts for 96 % of all highly oxygenated acyl RO2 signals. Considering that the acyl RO2 with small molecular size are generally the ring-opened RO2, the autoxidation rate constant of their precursor RO2 is expected to be relatively high (e.g., 1 s−1) (Iyer et al., 2021). Taking a RO2 cross-reaction rate constant of (Zhao et al., 2018) and a model-predicted total RO2 concentration of 1.7 ppb (Exp 8), the simulated contributions of autoxidation and cross-reactions to the total RO2 reaction are 96.0 % and 4.0 %, respectively. Considering a RO2 cross-reaction rate constant that is 10 times larger (i.e., ), the simulated contributions of RO2 autoxidation and cross-reactions would be 70.4 % and 29.6 %, respectively. These simulations suggest that the autoxidation of aldehydic RO2 plays a dominant role in the formation of the highly oxygenated acyl RO2. Although the acyl RO2 with low oxygen content were not measured in this study, all acyl RO2 containing oxygen atoms less than 6 seem to be derived from the cleavage of the C–C bond or H shift of RO containing an α-ketone or aldehyde in the currently known reaction mechanisms (Figs. 4 and S11).
Recently, Shen et al. (2022) found that the hydrogen abstraction by OH radicals during α-pinene oxidation plays an important role in HOM formation. In such mechanisms, the primary RO2 reacts with NO and forms RO radicals, which could undergo rapid ring-breaking reactions to form a series of ring-opened C10H15Ox-RO2, which contains aldehyde functionality and can easily autoxidize to C10 acyl RO2. In the absence of NO, the cross-reactions of RO2 can also produce RO radicals. However, only a few C10 acyl RO2 were detected in this study, and they contribute less than 1 % of the total C10 RO2 signal. This phenomenon could be due to the fact that the primary RO2 (C10H15O2) formed by the hydrogen abstraction by OH radical are least oxidized with only 2 oxygen atoms, which are expected to have a relatively low cross-reaction rate constant (Orlando and Tyndall, 2012; Berndt et al., 2018). As a result, the formation of ring-opened C10H15Ox-RO2 via cross-reactions of the primary C10H15O2-RO2 may not be important. As shown in Fig. 6, when the cross-reaction rate constants of C10H15O2-RO2 are considered to be , the simulated contribution of the H-abstraction pathway to the HOM formation is less than 3 % under both low-reacted (2.4 ppb) and high-reacted (9.6 ppb) α-pinene conditions. It should be note that the cross-reaction rate constants of the less-oxygenated RO2 could be even lower (Orlando and Tyndall, 2012); therefore the contribution of this pathway to HOM formation could be ignored when NO is absent.
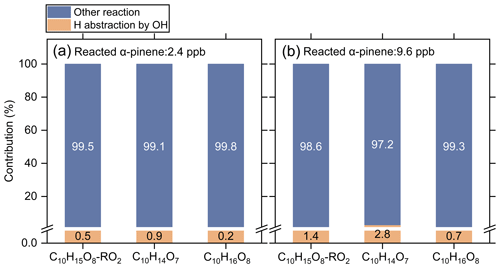
Figure 6Contributions of the H-abstraction pathway by OH radicals (yellow) and OH addition and ozonolysis pathways (blue) to the formation of typical HOMs under low-reacted (a) and high-reacted (b) α-pinene conditions simulated by the kinetic model. The cross-reaction rate constant was set to for the primary C10H15O2-RO2 and for the more oxygenated RO2.
In the presence of cyclohexane as an OH scavenger (Fig. S12, Exp 32), the measured signals of C10H17Ox-RO2 formed via the OH addition channel and the corresponding C10H18Ox HOMs decrease by more than 70 %, while the C10H15Ox-RO2 and its related closed-shell products decrease by less than 15 %, in good agreement with the measurements in previous studies (Zhao et al., 2018). As the C10H16O8 HOM could come from both C10H15Ox-RO2 and C10H17Ox-RO2, its reduction is at a medium level. The significantly smaller decrease in the signals of C10H15Ox-RO2 and its corresponding closed-shell products as compared to those of C10H17Ox-RO2 and the related closed-shell products further illustrates that the H abstraction by OH has a minor contribution to HOM formation in the absence of NO.
Figure 7 shows the changes in measured signal of C10H15O8-RO2 and C10H15O10-RO2 as a function of initial NO concentration (Exps 33–56). It should be noted that due to the existence of O3 in our experiments, these two RO2 could come from both O3 and OH reactions with α-pinene, and NO could be rapidly oxidized to NO2 by O3. The normalized signals of C10H15O8-RO2 and C10H15O10-RO2 increase firstly under low NO conditions, which is similar to the change of acyl RO2 as shown in Fig. 5. This increase could be for two reasons: (1) the promoted formation of C10H15O8 and C10H15O10 acyl RO2 from the H-abstraction channel by NO addition and (2) the equilibrium decomposition of ROONO2 formed by the two alkyl RO2 from ozonolysis of α-pinene in the chemical ionization inlet (see Sect. 3.1). As mentioned above, the ring-opened C10H15Ox-RO2 formed from the H-abstraction channel contain aldehyde functionality and can autoxidize rapidly. The F0AM simulations show that the C10H15O8 and C10H15O10 acyl RO2 formed from the H-abstraction channel contribute to 68 % and 56 % of the total C10H15O8-RO2 and C10H15O10-RO2 with the addition of 10 ppb NO, respectively. Therefore, the initial increases of these two RO2 with increasing NO concentration are likely mainly due to the enhanced formation of C10H15O8 and C10H15O10 acyl RO2. When the NO concentration increases to a high level, there is more NO and NO2 in the system, which promotes the consumption of acyl RO2. As a result, C10H15O8-RO2 exhibits a decreasing trend, and the increasing extend of C10H15O10-RO2 becomes much smaller.
3.3 Contributions of acyl RO2 to the formation of gas-phase HOMs
With the addition of NO2, the distribution of gas-phase products in the α-pinene ozonolysis changes significantly (see Fig. 1), and the consumption of acyl RO2 by NO2 plays an important role. NO2 influences the formation of HOM monomers mainly in three ways. Firstly, NO2 could react rapidly with acyl RO2 and form RC(O)OONO2, thus inhibiting the formation of HOMs with the involvement of acyl RO2. Secondly, as mentioned above, although ROONO2 is thermally unstable, their formation–decomposition equilibrium still consumes a small amount of alkyl RO2, resulting in a decrease in HOM formation. Thirdly, NO2 can consume a part of HO2 radicals (Fig. S13), thus inhibiting the RO2+HO2 reaction pathway.
Figure 8 shows the normalized signal of C7–C10 HOM monomers as a function of initial NO2 concentration. The signals of C7, C8, and some of C9 HOMs decrease significantly with increasing NO2 concentration due to the relatively large contribution of acyl RO2 to the total C7–C9 RO2. The C7 HOMs decrease by more than 50 % when the NO2 concentration reaches 30 ppb, while C8 HOMs decrease by more than 70 %, and some of them even decrease by 90 %. The C9 HOMs decrease by 30 %–60 %, and the species with relatively large decrease are mostly acyl RO2-related HOMs. For C10 HOMs, although there is also an obvious decrease in their formation with the addition of NO2, most of them have a smaller decreasing extent compared to the C7–C9 HOMs due to the low contribution of acyl RO2 to the C10 RO2. It is worth noting that a few C10 HOMs increase initially with the addition of NO2 up to 10 ppb, suggesting that there might be some processes that promote the formation of their precursor RO2 radicals and thus offset the inhibiting effect of NO2.
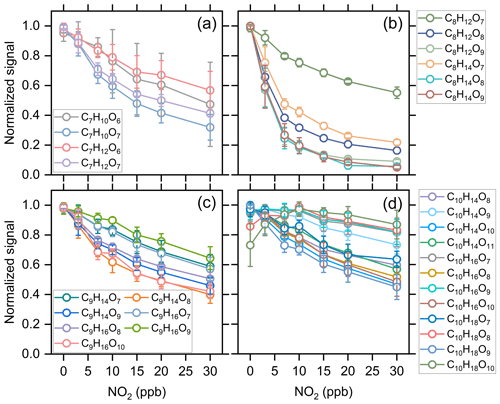
Figure 8Averaged normalized signal of the measured C7–C10 HOMs as a function of the added NO2 concentration (Exps 1–28).
As mentioned above, the addition of NO2 has the most significant influence on the formation of small HOM monomers. Combined with the large contribution (67 %–94 %) of acyl RO2 to the total C7 and C8 RO2 (Fig. 3), it can be considered that the reduction in the formation of C7 and C8 HOM monomers with NO2 addition is overwhelmingly due to the consumption of acyl RO2 by NO2. As a result, acyl RO2 were found to have a contribution of 50 %–90 % to C7 and C8 HOM monomer formation during α-pinene ozonolysis. Since acyl RO2 also have a considerable contribution (32 %) to the total C9 RO2, an upper limit (30 %–60 %) of their contribution to C9 HOMs could be derived with the assumption that the decrease of C9 HOMs with the addition of NO2 is also mainly due to the consumption of C9-acyl RO2 by NO2. By contrast, acyl RO2 account for a very small fraction (0.4 %) of the total C10 RO2, and their contribution to C10 HOMs cannot be quantified based solely on the experimental measurements given that the equilibrium reaction between alkyl RO2 and NO2 can also affect the formation of HOMs. Therefore, we used F0AM to simulate the contribution of acyl RO2 to C10 HOM formation according to the acyl RO2 measured in this study. It should be noted that the HOMs from the acyl RO2 and its subsequent RO2 (formed from acyl RO2 reactions) are all considered to be acyl-RO2-related HOMs in the model.
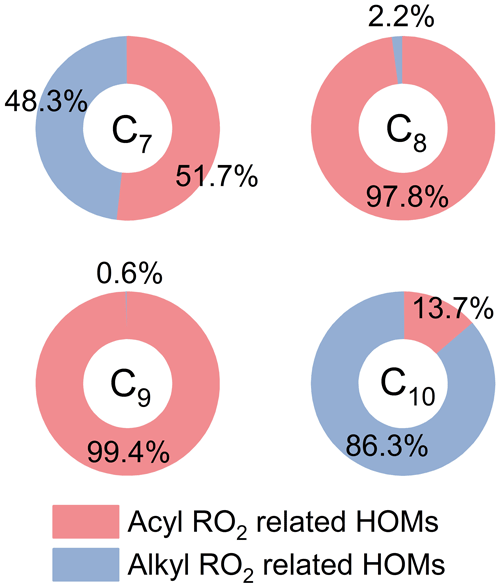
Figure 9Simulated average contribution of acyl and alkyl RO2 to C7–C10 HOM formation from ozonolysis of α-pinene under typical experimental conditions (Exps 1, 8, 15, and 22).
As mentioned above, the formation of ring-opened C10H15O4-RO2 reported by Iyer et al. (2021) is included in the model, and its autoxidation produces a ring-opened acyl C10H15O8-RO2. When we consider the upper limit of the yield of ring-opened C10H15O4-RO2 (89 %) in the model and assume that the other primary RO2 with the cyclobutyl ring autoxidize at a very slow rate (0.01 s−1), the simulated acyl C10H15O8-RO2 would contribute to ∼80 % of the total C10 RO2. However, we could not see a large decrease in the measured signal of C10H15O8-RO2 and its related HOM monomers with the addition of NO2. Similarly, a recent study by Zhao et al. (2022) found that the C10H15O8-related monomers and dimers in α-pinene SOA did not significantly decrease with NO2 addition. There might be three reasons for the discrepancy between the simulations and measurements. Firstly, the yield of the ring-opened C10H15O4-RO2 might be significantly smaller than 89 % (Zhao et al., 2021; Meder et al., 2023). Secondly, the autoxidation rate of other primary C10H15O4-RO2 with the cyclobutyl ring could be significantly larger than 0.01 s−1. Thirdly, the ring-opened C10H15O8-RO2, a highly functionalized acyl RO2 radical with an –OOH group, may be able to undergo very fast intramolecular H-scrambling reactions to form a peroxy acid as suggested by Knap and Jørgensen (2017), which would compete with the NO2 reaction and result in a lower reduction in its signal upon NO2 addition (see details in Sect. S3).
To examine the contributions of acyl RO2 to C10 HOM production, we updated the branching ratios and autoxidation rates of the primary RO2 during α-pinene ozonolysis in the model according to the recent studies (Kurten et al., 2015; Claflin et al., 2018; Zhao et al., 2021; Berndt, 2022) (Table S3) and used a lower limit (30 %) of the ring-opened C10H15O4-RO2 yield reported by Iyer et al. (2021). As displayed in Fig. 9, the simulated acyl RO2-related HOMs contribute to 14 % of the total C10 HOMs, which is slightly smaller than the measured decrease in C10 HOMs with the addition of NO2. This discrepancy could be for two reasons. Firstly, the decrease in HOMs can partly result from the consumption of alkyl RO2 and HO2 radicals by the addition of NO2. Secondly, as mentioned above, there might be other C10 acyl RO2 that were not observed in this study due to the decomposition of the ROONO2 from the alkyl RO2 with the same formulas. The contributions of acyl RO2 to the formation of C7–C9 HOMs were also simulated (Fig. 9). For C7 and C8 HOMs, the model predicts a contribution of 52 %–98 % from acyl RO2, which is consistent with the measurements (50 %–90 %). However, the simulated contribution of acyl RO2 to C9 HOMs is over 99 %, which is not consistent with the measurements (Fig. 8c). Recent studies indicated that the CI radicals from α-pinene ozonolysis did not form the alkyl C9H15O3-RO2 (C96O2 in default MCM v3.3.1) (Kurten et al., 2015; Zhao et al., 2021; Berndt, 2022). As a result, this primary C9 alkyl RO2 was not considered in the model, and most of C9 RO2 considered are acyl RO2 or from acyl RO2 reactions. In view of the significantly lower measured (less than 30 %–60 %) than simulated (over 99 %) contribution of acyl RO2 to C9 HOMs, we speculate that a small part of CI radicals might be able to form the C9H15O3-RO2, which could further react to form highly oxygenated alkyl C9 RO2.
A sensitivity analysis of the alkyl C9H15O3-RO2 yield was conducted to see its influence on the contribution of acyl RO2 to the total C9 HOMs. The model simulations show that when the yield of this C9 RO2 from one of the CIs ranges between 0.5 % and 2 %, the contribution of acyl RO2 to the total C9 HOMs ranges from 27.5 % to 59.8 % (Fig. S15), which is almost consistent with the measurements. This result indicates that a small part of CIs could generate the C9 alkyl RO2. We note that the small production of C9H15O3-RO2 from CIs has no significant influence on the yield of C10H15O4-RO2 and the subsequent acyl RO2. As shown in Fig. S16, as the C9H15O3-RO2 yield increases from 0 % to 3 %, the simulated concentrations of C10H15O4-RO2 exhibit negligible to small (5 %) changes. As the C9H15O3-RO2 is considered to only produce highly oxygenated alkyl RO2 in the model, it results in a decrease in the contribution of acyl RO2 to the total C9 HOMs. However, the contributions of acyl RO2 to total C7, C8, and C10 HOMs are almost unchanged.
The cross-reaction rate constant of acyl RO2 is generally larger than that of alkyl RO2 (Atkinson et al., 2007; Orlando and Tyndall, 2012), and the fast cross-reaction may lead to an important contribution to the HOM dimer production. The responses of dimer formation to increasing concentration of initial NO2 during α-pinene ozonolysis are given in Fig. 10. The C14–C18 dimers decrease by up to 50 %–95 % with the increase in NO2 concentration up to 30 ppb (Fig. 10a–e). The rapid cross-reaction rate of acyl RO2, as well as their dominant contribution to the small RO2 species, makes acyl RO2 an important contributor to the formation of these dimers. The consumption of acyl RO2 by NO2 greatly inhibits the bimolecular reactions involving acyl RO2, resulting in a rapid decrease in the signal of the corresponding dimers. Considering the predominance of acyl RO2 in small RO2 and their high reaction rate with NO2 compared to the alkyl RO2, it can be concluded that the cross-reactions involving acyl RO2 contribute to roughly 50 %–95 % of the C14–C18 dimer formation.
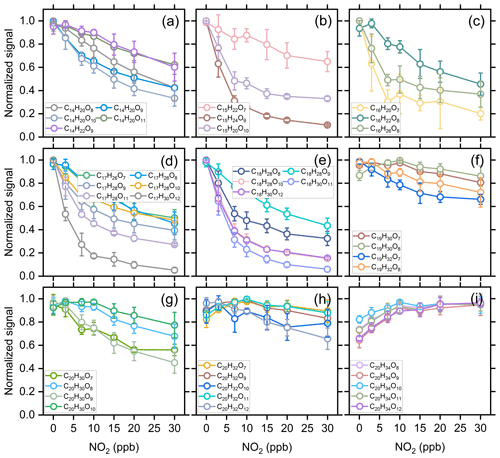
Figure 10Averaged normalized signal of the measured C14–C20 dimers as a function of the added NO2 concentration (Exps 1–28).
For C19 dimers, due to the relatively smaller contribution of acyl RO2 to C9 and C10 RO2, their signal decreases only by 10 %–40 %, and this reduction has contributions from both acyl and alkyl RO2. For C20 dimers, their signal changes with the addition of NO2 can be discussed according to the number of hydrogen atoms in the molecules. Firstly, the signals of C20H30O7 and C20H30O9 decrease by 40 %–60 % with the addition of 30 ppb NO2, indicating a significant contribution of acyl RO2 such as C10H15O5-RO2 (acyl RO2 in default MCM v3.3.1) and C10H15O7-RO2 in their formation, while other C20H30Ox dimers decrease by ∼30 %. The C20H32Ox dimer series also exhibits a small reduction (less than 20 %) with the addition of NO2. However, the C20H34Ox series shows an unexpected increase with the addition of NO2 up to 10 ppb and almost remains unchanged with the further increase in NO2 concentration. Given that the cross-reaction rate constant of acyl RO2 can be orders of magnitude higher than that of counterpart alkyl RO2 (Atkinson et al., 2007; Orlando and Tyndall, 2012), the rapid consumption of acyl RO2 by NO2 would preserve the alkyl RO2 that tend to react with acyl RO2 at a fast rate in the absence of NO2, which to some extent would elevate the concentration of alkyl RO2 in the system and thus promote the less competitive alkyl RO2 + alkyl RO2 reactions to form C20H34Ox dimers. The slight increase in some C10H18Ox HOMs with the addition of NO2 up to 10 ppb could also be for this reason.
According to the noticeable increasing trend in C20H34Ox as compared to other C20 dimers, we speculate that acyl RO2 react faster with C10H17Ox alkyl RO2 than with C10H15Ox alkyl RO2. Therefore, when the acyl RO2 are depleted, the preservation of C10H17Ox-RO2 is more significant, and the promotion of their cross-reactions to form C20H34Ox is more evident. It is also possible that the reaction of NO2 with C10H17Ox alkyl RO2 is less efficient compared to the reaction with C10H15Ox alkyl RO2, so more C10H17Ox than C10H15Ox is available for dimer formation in the presence of NO2.
To further prove the above two speculations, we performed sensitivity analyses for the reaction rates of C10H17Ox-RO2 using F0AM. Figure 11a shows the changes in C20H34Ox dimers with NO2 addition at different C10H17Ox-RO2 + NO2 reaction rates under the conditions of Exps 8–14. As the reaction rate varies from to , the increasing trend of C20H34Ox dimers versus the added NO2 concentration is weakened, and the simulation is more deviated from the measurements. When the reaction rate increases to , the C20H34Ox dimers decrease significantly with increasing NO2, which is in striking contrast to the measurements. Figure 11b presents the sensitivity analysis results for the cross-reaction rate constants of acyl RO2 + C10H17Ox-RO2. As this rate constant varies from to , the increasing trend of C20H34Ox versus the NO2 concentration is more pronounced and more consistent with the measurements. These sensitivity analyses support our speculation that the C10H17Ox alkyl RO2 may be different from other alkyl RO2 radicals in terms of the reaction efficiency with NO2 and acyl RO2 species, which leads to different responses of C20H34Ox dimers to NO2 addition compared to other C20 dimers. These results also suggest that the presence of acyl RO2 could affect the fate and contribution of alkyl RO2 to HOM formation in atmospheric oxidation systems given the different reactivity of acyl RO2 from alkyl RO2.
In this study, the molecular identities, formation mechanisms, and contributions of acyl RO2 to the formation of HOMs during ozonolysis of α-pinene are investigated using a combination of flow reactor experiments and detailed kinetic model simulations. Based on the marked decrease in RO2 signal as a function of initial NO2 concentration, a total of 10 highly oxygenated acyl RO2 are identified during α-pinene ozonolysis. The acyl RO2 contribute to 67 %, 94 %, and 32 % of C7, C8, and C9 highly oxygenated RO2 but only 0.4 % of C10 highly oxygenated RO2, respectively, when NO is absent. Three main pathways are identified for the formation of monoterpene-derived acyl RO2: (i) the autoxidation of RO2 containing aldehyde groups, (ii) the cleavage of a C–C bond of RO containing an α-ketone group, and (iii) the intramolecular H shift of RO containing an aldehyde group. The autoxidation of aldehydic RO2 formed involving multiple RO decomposition or ring-opening steps plays a dominant role in the formation of the highly oxygenated acyl RO2 radicals (oxygen atom number ≥6), while the less-oxygenated acyl RO2 (oxygen atom number <6) are mainly derived from the other two pathways.
The acyl-RO2-involved reactions explain 50 %–90 % of C7 and C8 HOM monomers and 14 % of C10 HOMs, respectively. For C9 HOMs, this contribution can be up to 30 %–60 %. For the HOM dimers, acyl-RO2-involved reactions contribute 50 %–95 % to the formation of C14–C18 dimers. Owing to the higher cross-reaction rate constant of acyl RO2 compared to alkyl RO2, the acyl RO2 + alkyl RO2 reaction would outcompete the alkyl RO2 + alkyl RO2 reaction. Therefore, the rapid consumption of acyl RO2 by NO2 in the experiments (as well as in polluted atmospheres) would make the alkyl RO2 that are supposed to react with acyl RO2 retained, which to some extent elevates the concentration of alkyl RO2 in the system and thus promotes the reaction of alkyl RO2 + alkyl RO2 to form dimers such as C20H34Ox. The contribution of H abstraction of α-pinene by OH radical to the formation of acyl RO2 and HOMs is found to be negligible in the absence of NO. This is because the primary C10H15O2-RO2 radicals formed in such pathways are least-oxidized and thus have relatively low cross-reaction efficiency to produce RO radicals, which are the key intermediates for the formation of acyl RO2 and HOMs in that channel. However, in the presence of NO, the formation of highly oxygenated acyl RO2 via the H-abstraction pathway is demonstrated, consistent with previous studies (Shen et al., 2022).
In this study, acyl RO2 species are identified according to a dramatic decrease in their signals with the addition of NO2. It should be noted that the presence of NO2 could also inhibit the formation of alkyl RO2 species involving acyl RO2 reactions. If there are any contributions of alkyl RO2 to acyl RO2 identified in this study, the influence of such alkyl RO2 species on HOM formation would reflect an indirect effect of acyl RO2. However, given that the formation of most of the acyl RO2 identified in this study can be reasonably explained by the proposed mechanisms and verified by their responses to the addition of NO, the acyl RO2 identified here are expected to have no significant contributions from alkyl RO2. Currently, the reaction kinetics of monoterpene-derived acyl RO2 are still poorly understood. Considering the important contribution of acyl RO2 to HOM formation, further kinetic studies are needed to get more specific rate constants for their autoxidation and cross-reactions, thereby deepening our understanding of the role of acyl RO2 in HOM and SOA formation under atmospheric conditions.
The data presented in this work are available upon request from the corresponding author.
The supplement related to this article is available online at: https://doi.org/10.5194/acp-23-12691-2023-supplement.
YZ and HZ designed the study, and HZ, DH, and JZ performed the experiments. YZ and HZ analyzed the data, conducted model simulations, and wrote the paper. All other authors contributed to the discussion and writing.
The contact author has declared that none of the authors has any competing interests.
Publisher’s note: Copernicus Publications remains neutral with regard to jurisdictional claims made in the text, published maps, institutional affiliations, or any other geographical representation in this paper. While Copernicus Publications makes every effort to include appropriate place names, the final responsibility lies with the authors.
Yue Zhao acknowledges the Program for Professor of Special Appointment (Eastern Scholar) at Shanghai Institutions of Higher Learning.
This research has been supported by the National Natural Science Foundation of China (grant nos. 22022607, 21806104, and 42005090) and the Program for Professor of Special Appointment (Eastern Scholar) at Shanghai Institutions of Higher Learning.
This paper was edited by John Liggio and reviewed by two anonymous referees.
Atkinson, R., Hasegawa, D., and Aschmann, S. M.: Rate constants for the gas-phase reactions of O3 with a series of monoterpenes and related compounds at 296±2 K, Int. J. Chem. Kinet., 22, 871–887, https://doi.org/10.1002/kin.550220807, 1990.
Atkinson, R., Baulch, D. L., Cox, R. A., Crowley, J. N., Hampson, R. F., Hynes, R. G., Jenkin, M. E., Rossi, M. J., and Troe, J.: Evaluated kinetic and photochemical data for atmospheric chemistry: Volume III – gas phase reactions of inorganic halogens, Atmos. Chem. Phys., 7, 981–1191, https://doi.org/10.5194/acp-7-981-2007, 2007.
Bell, D. M., Wu, C., Bertrand, A., Graham, E., Schoonbaert, J., Giannoukos, S., Baltensperger, U., Prevot, A. S. H., Riipinen, I., El Haddad, I., and Mohr, C.: Particle-phase processing of α-pinene NO3 secondary organic aerosol in the dark, Atmos. Chem. Phys., 22, 13167–13182, https://doi.org/10.5194/acp-22-13167-20222, 2022.
Berndt, T.: Peroxy radical processes and product formation in the OH radical-initiated oxidation of α-pinene for near-atmospheric conditions, J. Phys. Chem. A, 125, 9151–9160, https://doi.org/10.1021/acs.jpca.1c05576, 2021.
Berndt, T.: Peroxy radical and product formation in the gas-phase ozonolysis of α-pinene under near-atmospheric conditions: occurrence of an additional series of peroxy radicals O,O–C10H15O(O2)yO2 with y=1–3, J. Phys. Chem. A, 126, 6526–6537, https://doi.org/10.1021/acs.jpca.2c05094, 2022.
Berndt, T., Richters, S., Jokinen, T., Hyttinen, N., Kurtén, T., Otkjær, R. V., Kjaergaard, H. G., Stratmann, F., Herrmann, H., and Sipilä, M.: Hydroxyl radical-induced formation of highly oxidized organic compounds, Nat. Commun., 7, 1–8, https://doi.org/10.1038/ncomms13677, 2016.
Berndt, T., Mentler, B., Scholz, W., Fischer, L., Herrmann, H., Kulmala, M., and Hansel, A.: Accretion product formation from ozonolysis and OH radical reaction of α-pinene: mechanistic insight and the influence of isoprene and ethylene, Environ. Sci. Technol., 52, 11069–11077, https://doi.org/10.1021/acs.est.8b02210, 2018.
Bianchi, F., Kurteìn, T., Riva, M., Mohr, C., Rissanen, M. P., Roldin, P., Berndt, T., Crounse, J. D., Wennberg, P. O., and Mentel, T. F.: Highly oxygenated organic molecules (HOM) from gas-phase autoxidation involving peroxy radicals: A key contributor to atmospheric aerosol, Chem. Rev., 119, 3472–3509, 2019.
Calvert, J. G., Derwent, R. G., Orlando, J. J., Wallington, T. J., and Tyndall, G. S.: Mechanisms of atmospheric oxidation of the alkanes, Oxford University Press, Oxford, 992 pp., ISBN: 978-0195365818, 2008.
Claflin, M. S., Krechmer, J. E., Hu, W., Jimenez, J. L., and Ziemann, P. J.: Functional group composition of secondary organic aerosol formed from ozonolysis of α-pinene under high VOC and autoxidation conditions, ACS Earth Space Chem., 2, 1196–1210, https://doi.org/10.1021/acsearthspacechem.8b00117, 2018.
Ehn, M., Thornton, J. A., Kleist, E., Sipilä, M., Junninen, H., Pullinen, I., Springer, M., Rubach, F., Tillmann, R., and Lee, B.: A large source of low-volatility secondary organic aerosol, Nature, 506, 476–479, https://doi.org/10.1038/nature13032, 2014.
Fry, J. L., Kiendler-Scharr, A., Rollins, A. W., Wooldridge, P. J., Brown, S. S., Fuchs, H., Dubé, W., Mensah, A., dal Maso, M., Tillmann, R., Dorn, H.-P., Brauers, T., and Cohen, R. C.: Organic nitrate and secondary organic aerosol yield from NO3 oxidation of β-pinene evaluated using a gas-phase kinetics/aerosol partitioning model, Atmos. Chem. Phys., 9, 1431–1449, https://doi.org/10.5194/acp-9-1431-2009, 2009.
Fry, J. L., Draper, D. C., Barsanti, K. C., Smith, J. N., Ortega, J., Winkler, P. M., Lawler, M. J., Brown, S. S., Edwards, P. M., and Cohen, R. C.: Secondary organic aerosol formation and organic nitrate yield from NO3 oxidation of biogenic hydrocarbons, Environ. Sci. Technol., 48, 11944–11953, https://doi.org/10.1021/es502204x, 2014.
Guenther, A. B., Jiang, X., Heald, C. L., Sakulyanontvittaya, T., Duhl, T., Emmons, L. K., and Wang, X.: The Model of Emissions of Gases and Aerosols from Nature version 2.1 (MEGAN2.1): an extended and updated framework for modeling biogenic emissions, Geosci. Model Dev., 5, 1471–1492, https://doi.org/10.5194/gmd-5-1471-2012, 2012.
Iyer, S., Rissanen, M. P., Valiev, R., Barua, S., Krechmer, J. E., Thornton, J., Ehn, M., and Kurten, T.: Molecular mechanism for rapid autoxidation in alpha-pinene ozonolysis, Nat. Commun., 12, 878, https://doi.org/10.1038/s41467-021-21172-w, 2021.
Jenkin, M. E., Young, J. C., and Rickard, A. R.: The MCM v3.3.1 degradation scheme for isoprene, Atmos. Chem. Phys., 15, 11433–11459, https://doi.org/10.5194/acp-15-11433-2015, 2015.
Jokinen, T., Sipilä, M., Richters, S., Kerminen, V. M., Paasonen, P., Stratmann, F., Worsnop, D., Kulmala, M., Ehn, M., and Herrmann, H.: Rapid autoxidation forms highly oxidized RO2 radicals in the atmosphere, Angew. Chem. Int. Edit., 53, 14596–14600, https://doi.org/10.1002/anie.201408566, 2014.
Junninen, H., Ehn, M., Petäjä, T., Luosujärvi, L., Kotiaho, T., Kostiainen, R., Rohner, U., Gonin, M., Fuhrer, K., Kulmala, M., and Worsnop, D. R.: A high-resolution mass spectrometer to measure atmospheric ion composition, Atmos. Meas. Tech., 3, 1039–1053, https://doi.org/10.5194/amt-3-1039-2010, 2010.
Kirchner, F., Thuener, L., Barnes, I., Becker, K., Donner, B., and Zabel, F.: Thermal lifetimes of peroxynitrates occurring in the atmospheric degradation of oxygenated fuel additives, Environ. Sci. Technol., 31, 1801–1804, https://doi.org/10.1021/es9609415, 1997.
Knap, H. C. and Jørgensen, S.: Rapid Hydrogen Shift Reactions in Acyl Peroxy Radicals, J. Phys. Chem. A, 121, 1470–1479, https://doi.org/10.1021/acs.jpca.6b12787, 2017.
Knopf, D. A., Pöschl, U., and Shiraiwa, M.: Radial diffusion and penetration of gas molecules and aerosol particles through laminar flow reactors, denuders, and sampling tubes, Anal. Chem., 87, 3746–3754, https://doi.org/10.1021/ac5042395, 2015.
Kristensen, K., Watne, Å. K., Hammes, J., Lutz, A., Petäjä, T., Hallquist, M., Bilde, M., and Glasius, M.: High-molecular weight dimer esters are major products in aerosols from α-pinene ozonolysis and the boreal forest, Environ. Sci. Tech. Let., 3, 280–285, 2016.
Kurten, T., Rissanen, M. P., Mackeprang, K., Thornton, J. A., Hyttinen, N., Jorgensen, S., Ehn, M., and Kjaergaard, H. G.: Computational study of hydrogen shifts and ring-opening mechanisms in alpha-pinene ozonolysis products, J. Phys. Chem. A, 119, 11366–11375, https://doi.org/10.1021/acs.jpca.5b08948, 2015.
Li, X., Chee, S., Hao, J., Abbatt, J. P. D., Jiang, J., and Smith, J. N.: Relative humidity effect on the formation of highly oxidized molecules and new particles during monoterpene oxidation, Atmos. Chem. Phys., 19, 1555–1570, https://doi.org/10.5194/acp-19-1555-2019, 2019.
Lin, C., Huang, R.-J., Duan, J., Zhong, H., and Xu, W.: Primary and secondary organic nitrate in northwest China: a case study, Environ. Sci. Tech. Let., 8, 947–953, https://doi.org/10.1021/acs.estlett.1c00692, 2021.
Meder, M., Peräkylä, O., Varelas, J. G., Luo, J., Cai, R., Zhang, Y., Kurtén, T., Riva, M., Rissanen, M., Geiger, F. M., Thomson, R. J., and Ehn, M.: Selective deuteration as a tool for resolving autoxidation mechanisms in α-pinene ozonolysis, Atmos. Chem. Phys., 23, 4373–4390, https://doi.org/10.5194/acp-23-4373-2023, 2023.
Mentel, T. F., Springer, M., Ehn, M., Kleist, E., Pullinen, I., Kurtén, T., Rissanen, M., Wahner, A., and Wildt, J.: Formation of highly oxidized multifunctional compounds: autoxidation of peroxy radicals formed in the ozonolysis of alkenes – deduced from structure–product relationships, Atmos. Chem. Phys., 15, 6745–6765, https://doi.org/10.5194/acp-15-6745-2015, 2015.
Molteni, U., Simon, M., Heinritzi, M., Hoyle, C. R., Bernhammer, A.-K., Bianchi, F., Breitenlechner, M., Brilke, S., Dias, A., Duplissy, J., Frege, C., Gordon, H., Heyn, C., Jokinen, T., Kürten, A., Lehtipalo, K., Makhmutov, V., Petäjä, T., Pieber, S. M., Praplan, A. P., Schobesberger, S., Steiner, G., Stozhkov, Y., Tomé, A., Tröstl, J., Wagner, A. C., Wagner, R., Williamson, C., Yan, C., Baltensperger, U., Curtius, J., Donahue, N. M., Hansel, A., Kirkby, J., Kulmala, M., Worsnop, D. R., and Dommen, J.: Formation of highly oxygenated organic molecules from α-pinene ozonolysis: chemical characteristics, mechanism, and kinetic model development, ACS Earth Space Chem., 3, 873–883, https://doi.org/10.1021/acsearthspacechem.9b00035, 2019.
Noziere, B., Kalberer, M., Claeys, M., Allan, J., D'Anna, B., Decesari, S., Finessi, E., Glasius, M., Grgic, I., and Hamilton, J. F.: The molecular identification of organic compounds in the atmosphere: state of the art and challenges, Chem. Rev., 115, 3919–3983, 2015.
Orlando, J. J. and Tyndall, G. S.: Laboratory studies of organic peroxy radical chemistry: an overview with emphasis on recent issues of atmospheric significance, Chem. Soc. Rev., 41, 6294–6317, https://doi.org/10.1039/C2CS35166H, 2012.
Otkjær, R. V., Jakobsen, H. H., Tram, C. M., and Kjaergaard, H. G.: Calculated hydrogen shift rate constants in substituted alkyl peroxy radicals, J. Phys. Chem. A, 122, 8665–8673, https://doi.org/10.1021/acs.jpca.8b06223, 2018.
Pye, H. O. T., Chan, A. W. H., Barkley, M. P., and Seinfeld, J. H.: Global modeling of organic aerosol: the importance of reactive nitrogen (NOx and NO3), Atmos. Chem. Phys., 10, 11261–11276, https://doi.org/10.5194/acp-10-11261-2010, 2010.
Atkinson, R., Aschmann, S M., and Pitts Jr., J. N.: Rate constants for the gas-phase reactions of the OH radical with a series of monoterpenes at 294 ± 1 K, Int. J. Chem. Kinet., 18, 287–299, https://doi.org/10.1002/kin.550180303,1986.
Shen, H., Vereecken, L., Kang, S., Pullinen, I., Fuchs, H., Zhao, D., and Mentel, T. F.: Unexpected significance of a minor reaction pathway in daytime formation of biogenic highly oxygenated organic compounds, Sci. Adv., 8, eabp8702, https://doi.org/10.1126/sciadv.abp8702, 2022.
Sindelarova, K., Granier, C., Bouarar, I., Guenther, A., Tilmes, S., Stavrakou, T., Müller, J.-F., Kuhn, U., Stefani, P., and Knorr, W.: Global data set of biogenic VOC emissions calculated by the MEGAN model over the last 30 years, Atmos. Chem. Phys., 14, 9317–9341, https://doi.org/10.5194/acp-14-9317-2014, 2014.
Tyndall, G., Cox, R., Granier, C., Lesclaux, R., Moortgat, G., Pilling, M., Ravishankara, A., and Wallington, T.: Atmospheric chemistry of small organic peroxy radicals, J. Geophys. Res.-Atmos., 106, 12157–12182, 2001.
Villenave, E. and Lesclaux, R.: Kinetics of the cross reactions of CH3O2 and C2H5O2 radicals with selected peroxy radicals, J. Phys. Chem. C, 100, 14372–14382, https://doi.org/10.1021/jp960765m, 1996.
Wang, Y., Zhao, Y., Li, Z., Li, C., Yan, N., and Xiao, H.: Importance of hydroxyl radical chemistry in isoprene suppression of particle formation from α-pinene ozonolysis, ACS Earth Space Chem., 5, 487–499, https://doi.org/10.1021/acsearthspacechem.0c00294, 2021.
Wolfe, G. M., Marvin, M. R., Roberts, S. J., Travis, K. R., and Liao, J.: The Framework for 0-D Atmospheric Modeling (F0AM) v3.1, Geosci. Model Dev., 9, 3309–3319, https://doi.org/10.5194/gmd-9-3309-2016, 2016.
Xu, L., Møller, K. H., Crounse, J. D., Otkjær, R. V., Kjaergaard, H. G., and Wennberg, P. O.: Unimolecular reactions of peroxy radicals formed in the oxidation of α-pinene and β-pinene by hydroxyl radicals, J. Phys. Chem. A, 123, 1661–1674, https://doi.org/10.1021/acs.jpca.8b11726, 2019.
Yao, M., Zhao, Y., Hu, M., Huang, D., and Yan, N.: Multiphase reactions between secondary organic aerosol and sulfur dioxide: kinetics and contributions to sulfate formation and aerosol aging, Environ. Sci. Tech. Let., 6, 768–774, https://doi.org/10.1021/acs.estlett.9b00657, 2019.
Zhang, H., Yee, L. D., Lee, B. H., Curtis, M. P., Worton, D. R., Isaacman-VanWertz, G., Offenberg, J. H., Lewandowski, M., Kleindienst, T. E., and Beaver, M. R.: Monoterpenes are the largest source of summertime organic aerosol in the southeastern United States, P. Natl. Acad. Sci. USA, 115, 2038–2043, https://doi.org/10.1073/pnas.1717513115, 2018.
Zhao, Y., Thornton, J. A., and Pye, H. O. T.: Quantitative constraints on autoxidation and dimer formation from direct probing of monoterpene-derived peroxy radical chemistry, P. Natl. Acad. Sci. USA, 115, 12142–12147, https://doi.org/10.1073/pnas.1812147115, 2018.
Zhao, Y., Yao, M., Wang, Y. Q., Li, Z. Y., Wang, S. Y., Li, C. X., and Xiao, H. Y.: Acylperoxy Radicals as Key Intermediates in the Formation of Dimeric Compounds in alpha-Pinene Secondary Organic Aerosol, Environ. Sci. Technol., 56, 14249–14261, https://doi.org/10.1021/acs.est.2c02090, 2022.
Zhao, Z. X., Zhang, W., Alexander, T., Zhang, X., Martin, D. B. C., and Zhang, H. F.: Isolating a-Pinene Ozonolysis Pathways Reveals New Insights into Peroxy Radical Chemistry and Secondary Organic Aerosol Formation, Environ. Sci. Technol., 55, 6700–6709, https://doi.org/10.1021/acs.est.1c02107, 2021.