the Creative Commons Attribution 4.0 License.
the Creative Commons Attribution 4.0 License.
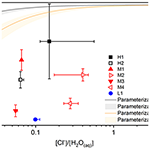
N2O5 uptake onto saline mineral dust: a potential missing source of tropospheric ClNO2 in inland China
Haichao Wang
Chao Peng
Xuan Wang
Shengrong Lou
Keding Lu
Guicheng Gan
Xiaohong Jia
Xiaorui Chen
Hongli Wang
Shaojia Fan
Xinming Wang
Nitryl chloride (ClNO2), an important precursor of Cl atoms, significantly affects atmospheric oxidation capacity and O3 formation. However, sources of ClNO2 in inland China have not been fully elucidated. In this work, laboratory experiments were conducted to investigate heterogeneous reactions of N2O5 with eight saline mineral dust samples collected from different regions in China, and substantial formation of ClNO2 was observed in these reactions. ClNO2 yields, φ(ClNO2), showed large variations (ranging from <0.05 to ∼0.77) for different saline mineral dust samples, depending on mass fractions of particulate chloride. In addition, φ(ClNO2) could increase, decrease or show insignificant change for different saline mineral dust samples when relative humidity (RH) increased from 18 % to 75 %. We further found that current parameterizations significantly overestimated φ(ClNO2) for heterogeneous uptake of N2O5 onto saline mineral dust. In addition, assuming a uniform φ(ClNO2) value of 0.10 for N2O5 uptake onto mineral dust, we used a 3-D chemical transport model to assess the impact of this reaction on tropospheric ClNO2 in China and found that weekly mean nighttime maximum ClNO2 mixing ratios could have been increased by up to 85 pptv during a severe dust event in May 2017. Overall, our work showed that heterogeneous reaction of N2O5 with saline mineral dust could be an important source of tropospheric ClNO2 in inland China.
- Article
(3764 KB) - Full-text XML
-
Supplement
(1654 KB) - BibTeX
- EndNote
The formation of O3 and secondary aerosols, two major air pollutants, is closely related to atmospheric oxidation processes (Lu et al., 2019). Primary pollutants emitted by natural and anthropogenic sources are oxidized by various oxidants to produce O3 and secondary aerosols, affecting air quality and climate. Major tropospheric oxidants include OH radicals, NO3 radicals and O3, and in the last 2 decades Cl atoms have been proposed as an important oxidant (Saiz-Lopez and von Glasow, 2012; Simpson et al., 2015; Wang et al., 2019). Rate constants for reactions of certain volatile organic compounds (VOCs) with Cl atoms can be a few orders of magnitude larger than those reacting with OH radicals (Atkinson and Arey, 2003; Atkinson et al., 2006); therefore, despite its lower concentrations in the troposphere, Cl can contribute significantly to atmospheric oxidation capacity in some regions (Saiz-Lopez and von Glasow, 2012; Simpson et al., 2015; Wang et al., 2019). For example, a modeling study (Sarwar et al., 2014) suggested that including Cl chemistry in the model could enhance oxidative degradation of VOCs by >20 % in some locations.
One major source of tropospheric Cl atoms is daytime photolysis of nitryl chloride (ClNO2) (Thornton et al., 2010; Simpson et al., 2015), which is formed in heterogeneous reaction of N2O5 with chlorine-containing particles (R1) at nighttime (Osthoff et al., 2008; Thornton et al., 2010):
The uptake coefficient, γ(N2O5), and the ClNO2 yield, φ(ClNO2), both depend on relative humidity (RH), aerosol composition and mixing state, etc. (Bertram and Thornton, 2009; Ryder et al., 2014; Mitroo et al., 2019; McNamara et al., 2020; Yu et al., 2020). Cl atoms produced by ClNO2 photolysis can effectively enhance atmospheric oxidation (Le Breton et al., 2018; Wang et al., 2019) and thus increase concentrations of O3 and OH radicals during the day (Simon et al., 2009; Riedel et al., 2014; Sarwar et al., 2014; Tham et al., 2016; Wang et al., 2016). In addition, ClNO2 is an important temporary reservoir of NOx at night and releases NO2 during the daytime via photolysis, thereby further affecting daytime photochemistry.
Sea spray aerosol is the most important source of particulate chloride (Cl−), and ClNO2 is expected to be abundant at marine and coastal regions impacted by anthropogenic emissions. High levels of ClNO2 have been observed at various marine and coastal regions across the globe (Simon et al., 2009; Riedel et al., 2012; Tham et al., 2014; Young et al., 2014; Wang et al., 2016; Osthoff et al., 2018; H. Wang et al., 2020; Yu et al., 2020). In addition, many studies (Thornton et al., 2010; Mielke et al., 2011; Phillips et al., 2012; Riedel et al., 2013; Bannan et al., 2015; Faxon et al., 2015; X. Wang et al., 2017; Z. Wang et al., 2017; Tham et al., 2018; Wang et al., 2018) have also reported significant amounts of ClNO2 at various continental sites with limited marine influence. For example, ClNO2 concentrations reached 4 ppbv in the summer on the North China Plain (Tham et al., 2016). These observations imply the importance of other sources for aerosol chloride, such as coal combustion (Eger et al., 2019), biomass burning (Ahern et al., 2017), waste incineration (Bannan et al., 2019) and snow-melting agent application (Mielke et al., 2016; McNamara et al., 2020).
In addition to insoluble minerals (e.g., quartz, feldspar, clay and carbonate), mineral dust aerosols emitted from saline topsoil in arid and semi-arid regions may contain significant amounts of soluble material such as chloride and sulfate (Gillette et al., 1992; Abuduwailli et al., 2008; Zhang et al., 2009; Wang et al., 2012; Jordan et al., 2015; Frie et al., 2017; Gaston et al., 2017; Tang et al., 2019; Gaston, 2020). As elemental and mineralogical compositions are different for conventional and saline mineral dust, they would differ significantly in their physicochemical properties and impacts on atmospheric chemistry and climate. For example, hygroscopicity and cloud condensation nuclei (CCN) activities of saline mineral dust can be much higher than those of conventional mineral dust (Pratt et al., 2010; Gaston et al., 2017; Tang et al., 2019; Zhang et al., 2020). Recent laboratory studies (Mitroo et al., 2019; Royer et al., 2021) have found that heterogeneous reactions of N2O5 with saline mineral dust originating from the western and southwestern USA can be very effective and produce significant amounts of ClNO2. Large variations in γ(N2O5) and φ(ClNO2) were reported (Mitroo et al., 2019; Royer et al., 2021), depending on RH as well as on the chemical and mineralogical contents of saline mineral dust samples.
A very recent study (Wu et al., 2020) showed that N2O5 uptake onto saline mineral dust contributed significantly to particulate nitrate formation during a dust storm event in Shanghai, China. One may further expect that it may have a profound effect on ClNO2, especially considering that vast areas in China are heavily affected by both mineral dust and NOx. Nevertheless, heterogeneous formation of ClNO2 from N2O5 uptake onto saline mineral dust in regions other than the USA has not been explored. In order to provide key parameters required to assess the potential of saline mineral dust as a ClNO2 source in China, we conducted a series of laboratory experiments to investigate ClNO2 formation in heterogeneous reaction of N2O5 with several saline mineral dust samples collected from different regions in China. In addition to differences in source regions, the saline mineral dust samples examined in our work have substantial variations in composition and mineralogy, enabling us to examine the effects of particle composition and water content on ClNO2 production. In order to better understand variations in ClNO2 yields with RH and samples, we experimentally measured mass hygroscopic growth factors of the eight samples examined, while previous studies (Mitroo et al., 2019; Royer et al., 2021) used the thermodynamic model ISORROPIA II (Fountoukis and Nenes, 2007) to predict particulate water contents. Based on our laboratory results, we further use a 3-D chemical transport model (GEOS-Chem) to assess the impacts of ClNO2 produced from N2O5 uptake onto mineral dust on ClNO2 and O3 in China during a major dust event which occurred in May 2017.
2.1 Characterization of saline mineral dust samples
Eight saline mineral dust samples, originating from five different provinces in northern China (including Ningxia, Xinjiang, Shandong, Inner Mongolia and Shaanxi), were examined in this work, and full information on these samples can be found elsewhere (Tang et al., 2019). Table 1 summarizes key information on these samples. According to their chloride contents, the eight samples were classified into three categories, including two high-chloride samples (H1 and H2), four medium-chloride samples (M1, M2, M3 and M4) and two low-chloride samples (L1 and L2).
Our previous work (Tang et al., 2019; Zhang et al., 2020) measured mass hygroscopic growth factors of the eight samples at 0 %–90 % RH with a RH resolution of 10 %, using a vapor sorption analyzer (Gu et al., 2017). As the highest RH at which heterogeneous reaction of N2O5 with saline mineral dust was conducted in our work was ∼75 %, we further measured mass growth factors of the eight samples at 75 %±2 % RH, and the results are also included in Table 1.
Table 1Overview of mass fractions of major soluble ions and mass ratios of particulate water at 75 %±2 % RH to dry particles for the eight saline mineral dust samples examined in this work. Mass fractions of major soluble ions were reported previously (Tang et al., 2019), and particulate water contents at 75 %±2 % RH were measured by the present work. The abbreviation n.d. denotes not detected.
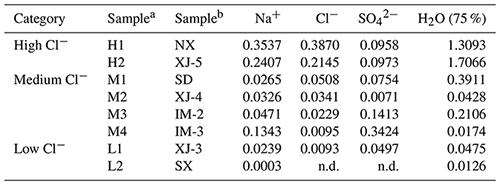
a Sample names used in the present work. b Corresponding sample names used in our previous work (Tang et al., 2019).
2.2 Experimental apparatus
Figure 1 shows the experimental apparatus used to study heterogeneous interactions of N2O5 with saline mineral dust. It mainly consists of three parts: (1) N2O5 generation, (2) gas–particle interaction, and (3) detection of N2O5 and ClNO2.
2.2.1 N2O5 generation
In our work, N2O5 was generated via oxidation of NO2 by O3. As shown in Fig. 1, a synthetic airflow (30 mL min−1) was passed over a Hg lamp to produce O3 via O2 photolysis at 184.95 nm. The photolysis module was stabilized at 35±0.2 ∘C using a Peltier cooler controlled by a proportion integration differentiation (PID) algorithm, in order to give stable O3 output. The O3/airflow was then mixed with a NO2 flow (80 mL min−1, 10 ppmv in synthetic air) in a temperature-stabilized polyfluoroalkoxy (PFA) reactor with a residence time of ∼70 s to produce N2O5. After exiting the PFA reactor, the flow (110 mL min−1) was then diluted with a humidified nitrogen flow (2500 mL min−1), and the RH of the humidified nitrogen flow was monitored using a hygrometer. The final flow had a total flow rate of 2610 mL min−1.
2.2.2 Heterogeneous interactions
As shown in Fig. 1, the mixed flow (2610 mL min−1) could be directed through a blank polytetrafluoroethylene (PTFE) membrane filter (47 mm, Whatman, USA) housed in a PFA filter holder, and in this case initial N2O5 and ClNO2 concentrations were measured; in our experiments, initial N2O5 concentrations were in the range of 0.4–1.0 ppbv. Alternatively, the flow could also be passed through a PTFE filter loaded with saline mineral dust, and thus N2O5 and ClNO2 concentrations after heterogeneous reaction with saline mineral dust loaded on the filter were measured. During our experiments, the flow could be switched back to pass through the blank filter in order to check whether the initial N2O5 and ClNO2 concentrations were stable.
Saline mineral dust particles were loaded onto PTFE filters using the method described in our previous study (Li et al., 2020; Jia et al., 2021). In brief, 10 mL of particle ethanol mixture was transferred onto a PTFE filter, and after ethanol was evaporated a relatively uniform particle film, as revealed by visual inspection, was formed on the filter. PTFE filters were weighted before and after being loaded with particles in order to determine the mass of particles loaded onto these filters. In our work, the mass of particles on filters was in the range of 0.6–7.3 mg.
2.2.3 Detection of N2O5 and ClNO2
After exiting one of the two filters, a flow of 2200 mL min−1 was sampled into a time-of-flight chemical ionization mass spectrometry (CIMS) instrument to measure N2O5 and ClNO2 concentrations, and the remaining flow (∼400 mL min−1) went into the exhaust. The CIMS instrument has been detailed previously (Kercher et al., 2009; Wang et al., 2016). In brief, N2O5 and ClNO2 were detected as I(N2O5)− and I(ClNO2)− clusters at 235 and 208 (R2a, R2b) using I− as the reagent ion, and a soft X-ray device (Hamamatsu, Soft X-Ray 120∘) was employed to generate I− from CH3I/N2. The CIMS instrument was calibrated before and after our experiments, which lasted for ∼1 month, and further details on calibration can be found in the Appendix. The detection limits were 2 pptv for N2O5 and 3 pptv for ClNO2, calculated as 4 times the standard deviation (4σ) when measuring blank samples with a 1 min average, and the accuracy was estimated to be ∼25 %.
2.3 Model description
We use GEOS-Chem (version 12.9.3) to quantify the effects of ClNO2 formation due to heterogeneous reaction of N2O5 with saline dust in China. The model, which includes a detailed representation of coupled ozone–NOx–VOC–aerosol–halogen chemistry (Wang et al., 2021), is driven by MERRA2 (the Modern-Era Retrospective Analysis for Research and Applications, Version 2) assimilated meteorological fields from the NASA Global Modeling and Assimilation Office (GMAO) with a native horizontal resolution of and 72 vertical levels from the surface to the mesosphere. Our simulation was conducted over East Asia (10∘ S–55∘ N, 60–150∘ E) at the native resolution with dynamical boundary conditions from a global simulation. Anthropogenic emissions in China are based on the Multi-resolution Emission Inventory for China (MEIC) (Zheng et al., 2018) and an inventory of HCl and fine particulate Cl− in China (Fu et al., 2018). Natural dust emissions are calculated based on Ridley et al. (2013). A more detailed description of the model and emissions can be found elsewhere (X. Wang et al., 2020).
For N2O5 uptake onto aqueous aerosols, the parameterization in our previous study (X. Wang et al., 2020) for γ(N2O5) and φ(ClNO2), which is based on a detail evaluation of different model parameterizations by previous work (McDuffie et al., 2018a, b), is used in this study, and more details can be found in the Supplement. For N2O5 uptake on dust aerosol, γ(N2O5) is always assumed to be 0.02, as recommended previously (Crowley et al., 2010; Tang et al., 2017), and φ(ClNO2) is assumed to be 0 in the standard case; i.e., no ClNO2 is produced in heterogeneous reaction of N2O5 with mineral dust.
Figure 2a shows changes in N2O5 and ClNO2 concentrations during an experiment in which heterogeneous reaction of N2O5 with sample H1 at 37 % RH was studied. As shown in Fig. 2a, when the mixed flow was passed through the blank filter (0–10 min), N2O5 concentrations were measured to be ∼350 pptv and ClNO2 was below the detection limit. The mixed flow was then passed through the particle-loaded filter at ∼10 min in order to initiate heterogeneous reaction of N2O5 with sample H1, and a significant decrease in N2O5 concentrations (from ∼350 to ∼150 pptv) and a significant increase in ClNO2 concentrations (from almost 0 to ∼150 pptv) were observed, suggesting that heterogeneous interaction with sample H1 substantially consumed N2O5 and generated ClNO2. In order to check if initial N2O5 and ClNO2 concentrations were stable, during our experiments the mixed flow was switched back to pass through the blank filter from time to time (e.g., at around 40, 75 and 105 min for the experiment displayed in Fig. 2a). Indeed, initial N2O5 and ClNO2 concentrations were constant in our experiments, with another two examples shown in Fig. 2b and c.
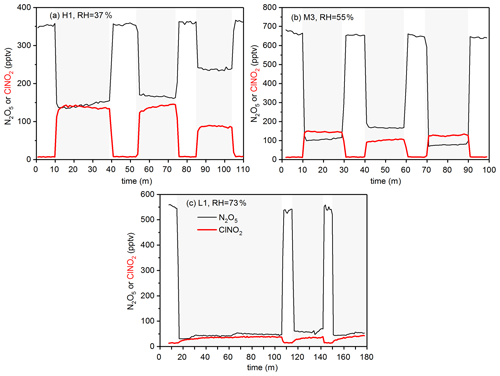
Figure 2Time series for measured N2O5 and ClNO2 concentrations after the mixed flow was passed through the blank filter or the particle-loaded filter: (a) H1, 37 % RH; (b) M3, 55 % RH; (c) L1, 73 % RH. Periods in which the mixed flow was passed through the particle-loaded filter are shaded gray.
Figure 2b and c show time series of measured N2O5 and ClNO2 concentrations in another two experiments, suggesting that heterogeneous reaction with sample M3 and L1 also led to substantial removal of N2O5. However, much less ClNO2 was produced for sample M3 and L1 when compared to sample H1 (Fig. 2a). The decrease in N2O5 concentrations, Δ[N2O5], and the increase in ClNO2 concentrations, Δ[ClNO2], can be used to calculate ClNO2 yields, φ(ClNO2), according to Eq. (1).
In this work we measured φ(ClNO2) for heterogeneous reaction of N2O5 with eight different saline mineral dust samples at four RH levels, and each experiment was repeated at least three times. It should be mentioned that during each experiment the measured φ(ClNO2) did not vary significantly with time, and therefore an average value of φ(ClNO2) was reported for each experiment. Table 2 summarizes measured φ(ClNO2) for the eight samples at different RH levels, and the results are further discussed in the following sections.
Table 2Measured ClNO2 yields for heterogeneous uptake of N2O5 onto saline mineral dust samples at different RH levels. All the errors given in this work are standard deviations. The uncertainty in RH was ±2 %.
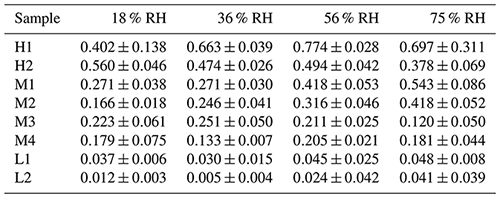
3.1 ClNO2 production yields
Figure 3 shows ClNO2 yields as a function of RH for the two samples with high chloride content (H1 and H2), and φ(ClNO2) values were found to be quite high for the two samples. To be more specific, the mass fraction of chloride was 0.3870 for sample H1, and φ(ClNO2) values were found to increase from 0.402±0.138 at 18 % RH to 0.774±0.028 at 56 % RH, and then they slightly decreased to 0.697±0.311 when RH was further increased to 75 %. For sample H2, the mass fraction of chloride (0.2145) was lower than for sample H1, and φ(ClNO2) showed a small decrease (or remained relatively constant) when RH was increased from 18 % to 56 %, ranging from 0.474±0.026 to 0.560±0.046; a further increase in RH to 75 % resulted in a small decrease in φ(ClNO2) to 0.378±0.069.
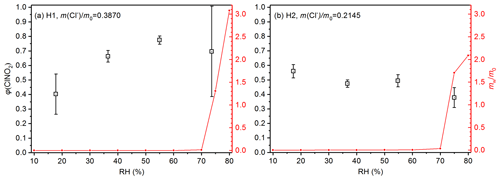
Figure 3Measured ClNO2 yields (black symbol) and (red line) as a function of RH for (a) H1 and (b) H2. The error bar represents standard deviation, and represents the normalized mass of particulate water (normalized to the mass of dry particles), which was measured as the relative increase in particle mass at a given RH compared to <1 % RH.
ClNO2 yields are shown in Fig. 4 as a function of RH for the two low-chloride samples (L1 and L2), and their mass fractions of chloride were <0.01. As shown in Fig. 4, φ(ClNO2) values were found to be always <0.05 for the two samples, suggesting that heterogeneous production of ClNO2 was very limited, despite substantial removal of N2O5 due to heterogeneous reaction (with an example shown in Fig. 2c). The low φ(ClNO2) values for sample L1 and L2 could be attributed to their low chloride contents. In addition, φ(ClNO2) appeared to increase with RH for L1 and L2; however, since the uncertainties associated with φ(ClNO2) were rather large for these two samples, the dependence of φ(ClNO2) on RH should be treated in caution.
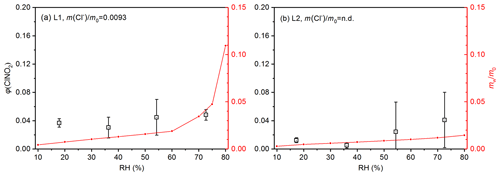
Figure 4Measured ClNO2 yields (black symbol) and (red line) as a function of RH for (a) L1 and (b) L2. The error bar represents standard deviation, and represents the normalized mass of particulate water (normalized to the mass of dry particles), which was measured as the relative increase in particle mass at a given RH compared to <1 % RH.
We also investigated ClNO2 production from heterogeneous reaction of N2O5 with four samples with medium-chloride contents (M1, M2, M3 and M4), and the results are displayed in Fig. 5. Mass fractions of chloride were determined to be 0.0508 for M1, 0.034 for M2, 0.0229 for M3 and 0.0095 for M4. ClNO2 yields were found to increase significantly with RH for M1 and M2; more specifically, φ(ClNO2) increased from 0.271±0.038 at 18 % RH to 0.543±0.086 at 75 % RH for sample M1 and increased from 0.166±0.018 at 18 % RH to 0.418±0.0052 at 75 % RH for sample M2. As shown in Fig. 5, the dependence of φ(ClNO2) on RH for the other two medium-chloride samples (M3 and M4) was rather different from that of M1 and M2. For sample M3, φ(ClNO2) first increased from 0.223±0.061 at 18 % RH to 0.251±0.050 at 36 % RH, and a further increase in RH to 75 % caused substantial reduction in φ(ClNO2). Finally, no significant variation in φ(ClNO2) with RH (18 %–75 %) was observed for sample M4.
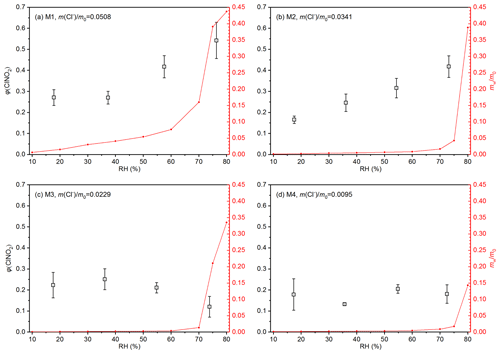
Figure 5Measured ClNO2 yields (black symbol) and (red line) as a function of RH for (a) M1, (b) M2, (c) M3 and (d) M4. The error bar represents standard deviation, and represents the normalized mass of particulate water (normalized to the mass of dry particles), which was measured as the relative increase in particle mass at a given RH compared to <1 % RH.
3.2 The effects of RH
The dependence of φ(ClNO2) on RH for the eight saline mineral samples we examined, as discussed in Sect. 3.1, exhibited two interesting features. First, when RH was as low as 18 %, large φ(ClNO2) values (>0.2) were observed for four samples (H1, H2, M1 and M3). As the deliquescence RH of NaCl is ∼75 %, one may wonder where aqueous chloride, which is necessary for heterogeneous formation of ClNO2, came from at 18 % RH. As initially suggested by a previous study (Mitroo et al., 2019), the occurrence of aqueous chloride in saline mineral dust particles at low RH could be due to the presence of CaCl2 and MgCl2, which were amorphous under dry conditions and could take up water at very low RH (Guo et al., 2019). Our previous study (Tang et al., 2019) measured water-soluble ions contained by the eight saline mineral dust samples, and as shown in Fig. S1 in the Supplement, the amounts of water-soluble Ca2+ in the four samples (H1, H2, M1 and M3) with larger φ(ClNO2) at 18 % RH were significantly larger than those in the other four samples (M2, M4, L1 and L2). This observation further supported our deduction that the presence of CaCl2 enabled efficient formation of ClNO2 at low RH.
The second interesting feature is that as shown in Figs. 3–5, φ(ClNO2) could increase, decrease or remain relatively constant with an increase in RH from 18 % to 75 %. This feature can be understood given the complex mechanisms driving heterogeneous uptake of N2O5 onto saline mineral dust (Mitroo et al., 2019; Royer et al., 2021): at a given RH, N2O5 can react with aqueous water, aqueous chloride and insoluble minerals, and only its reaction with aqueous chloride would produce ClNO2. The possible effects of RH on φ(ClNO2) are discussed below: (1) as RH increases, heterogeneous reactivity of N2O5 towards insoluble minerals can be enhanced, suppressed or remain largely unchanged (Tang et al., 2012, 2017); (2) an increase in RH would lead to further hygroscopic growth and dilution of aqueous solutions, leading to a decrease in φ(ClNO2) in this regard; (3) the increase in particulate water with RH would cause more chloride to be dissolved into aqueous solutions, and in this regard an increase in RH would promote ClNO2 formation. As a result, it is not surprising to observe different levels of dependence of φ(ClNO2) on RH for different saline mineral dust samples.
3.3 Discussion
Figure 6 shows the dependence of φ(ClNO2) on mass fractions of chloride for the eight samples we examined at four different RH levels. These samples showed significant variation in φ(ClNO2), ranging from <0.1 to >0.7, and φ(ClNO2) values were the largest for the two high-chloride samples (H1 and H2), followed by the medium-chloride samples (M1, M2, M3 and M4) and low-chloride samples (L1 and L2). Overall, a positive dependence of φ(ClNO2) on mass fractions of chloride was observed at each RH level. Figure 6 also reveals that the measured φ(ClNO2) values were very sensitive to mass fractions of chloride when the mass fractions of chloride were below 10 %. This is because an increase in RH would promote dissolution of chloride to aqueous water and thus enhance ClNO2 formation. However, as shown in Fig. 6, higher chloride contents did not always mean larger φ(ClNO2), and similar observations have also been reported in previous work (Mitroo et al., 2019; Royer et al., 2021).
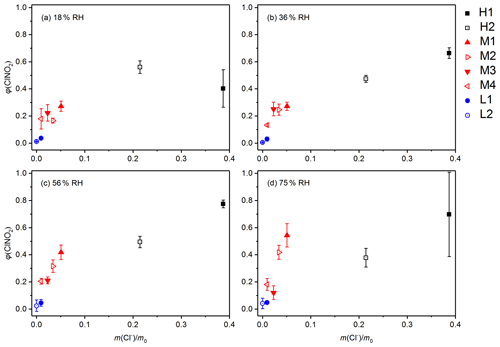
Figure 6Dependence of ClNO2 yields on mass fractions of chloride for the eight saline mineral dust samples at a given RH: (a) 18 % RH, (b) 36 % RH, (c) 56 % RH and (d) 75 % RH.
Two parameterizations have been widely used to predict the dependence of φ(ClNO2) on the chemical compositions and water contents of aqueous aerosol particles (Bertram and Thornton, 2009; Yu et al., 2020). Based on laboratory results, Bertram and Thornton (2009) suggested that ClNO2 yields can be calculated using Eq. (2):
where is the ratio of molar concentration of H2O to that of Cl− in aqueous particles, and the value of was suggested to be (Bertram and Thornton, 2009). Very recently, Yu et al. (2020) examined uptake coefficients of N2O5 onto ambient aerosol particles at four different sites in China and suggested that using a value of for would lead to better agreement between the measured and predicted uptake coefficients of N2O5 (Yu et al., 2020).
The two parameterizations were used in our work to calculate φ(ClNO2) at 75 % RH for the eight saline mineral dust samples we examined. was calculated from the measured mass growth factors at 75 % RH and the mass fractions of chloride, assuming that all the chloride contained by saline mineral dust samples was dissolved into aqueous solutions at 75 % RH. The comparison between measured and calculated φ(ClNO2) is displayed in Fig. 7, suggesting that both parameterizations significantly overestimated the measured φ(ClNO2) for all the eight saline mineral dust samples we investigated. A previous study (Mitroo et al., 2019) investigated φ(ClNO2) for heterogeneous uptake of N2O5 onto saline mineral dust samples collected in the southwestern USA, and similarly they found that the measured φ(ClNO2) values were significantly smaller than those predicted using the parameterization proposed by Bertram and Thornton (2009).
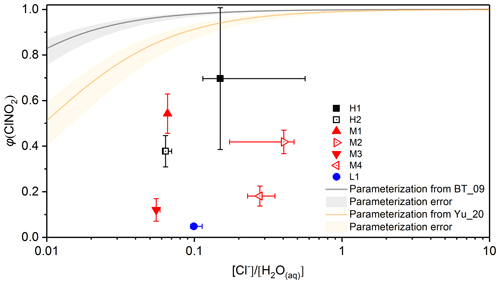
Figure 7Measured and calculated values of φ(ClNO2) at 75 %±2 % RH as a function of . Black and orange curves represent φ(ClNO2) calculated using the BT_09 parameterization (Bertram and Thornton, 2009) and the Yu_20 parameterization (Yu et al., 2020), and the associated errors are represented by the corresponding shading.
The observed discrepancies between measured and predicted φ(ClNO2) can be caused for several reasons. First, even at ∼75 % RH (the highest RH at which our experiments were conducted), chloride contained in saline mineral dust may not be fully dissolved, and therefore our calculation may overestimate and thus also overestimate φ(ClNO2). This effect should not be large as significant water uptake was observed at ∼75 % RH for the saline mineral dust sample we examined (Figs. 3–5). Second, perhaps more importantly, saline mineral dust samples contain substantial quantities of insoluble minerals, and some of these minerals, such as clays, are very reactive towards N2O5 (Tang et al., 2017), and only nitrate, and no ClNO2, was formed (Seisel et al., 2005; Karagulian et al., 2006; Tang et al., 2012). However, the two parameterizations did not take into account heterogeneous reaction of N2O5 with insoluble minerals and as a result would inevitably overestimate φ(ClNO2). Finally, our calculations assumed internal mixing, but inter- and intra-particle heterogeneity of saline mineral dust particles could also contribute to the observed gap between measured and calculated φ(ClNO2). For example, a wintertime field campaign at Ann Arbor (Michigan, USA) (McNamara et al., 2020) showed that due to nonhomogeneous chloride distribution across road salt aerosol particles, observed φ(ClNO2) values were significantly smaller than predicted values. The comparison between measured and predicted φ(ClNO2) suggests that while heterogeneous uptake of N2O5 onto saline mineral dust could be an important source of inland ClNO2, underlying mechanisms which affect heterogeneous production of ClNO2 from saline mineral dust have not been well elucidated.
We consider ClNO2 formation in heterogeneous uptake of N2O5 onto dust aerosol in GEOS-Chem to explore its atmospheric implications. Since Cl− concentration in mineral dust is not well known and currently we are not able to parameterize φ(ClNO2) for mineral dust (as discussed in Sect. 3.3), we use a fixed φ(ClNO2) value of 0.1 in our simulation. This value, which is at the low end of our measured range of φ(ClNO2) (<0.05 to ∼0.77), is higher than those values determined in our work for low-chloride samples but lower than those for medium-chloride samples. The purpose of our modeling work is to preliminarily assess whether N2O5 uptake onto saline dust may have important effects on tropospheric chemistry as a potential source of ClNO2. We focus on simulations on 2–7 May 2017, during which a large dust event took place in East Asia. It caused high concentrations of dust aerosols with a maximum hourly concentration higher than 1000 µg m−3 over a wide area in China (Zhang et al., 2018), which are also well captured by our simulations (Fig. S2 in the Supplement).
Figure 8 shows the weekly mean values of the nighttime maximum surface ClNO2 mixing ratios and the contribution of heterogeneous reaction of N2O5 with dust aerosol to ClNO2 over 2–7 May 2017. The impact of N2O5 uptake onto dust aerosol is calculated as the difference between the standard case in which φ(ClNO2) is assumed to be 0 for N2O5 uptake onto dust aerosol and the case in which φ(ClNO2) is assumed to be 0.1. Due to large diurnal variations and near-zero mixing ratios of ClNO2 in the daytime, we use the mean nighttime maximum value for ClNO2, following previous standard practice (Wang et al., 2019). The largest impact on ClNO2 is found in central China, where weekly mean nighttime maximum surface ClNO2 mixing ratios are increased by 85 pptv, due to the heavy impact of dust aerosol transported from the north and high NOx emissions in this region. Even larger effects (up to a 240 pptv increase in ClNO2) can be found on some individual days, as shown in Figs. S3 and S4 in the Supplement. These results suggest that N2O5 uptake onto dust could be an important source for tropospheric ClNO2 over central and northeast China, where ClNO2 formation is conventionally believed to be limited due to relatively low aerosol chloride levels from sea salts and anthropogenic sources.
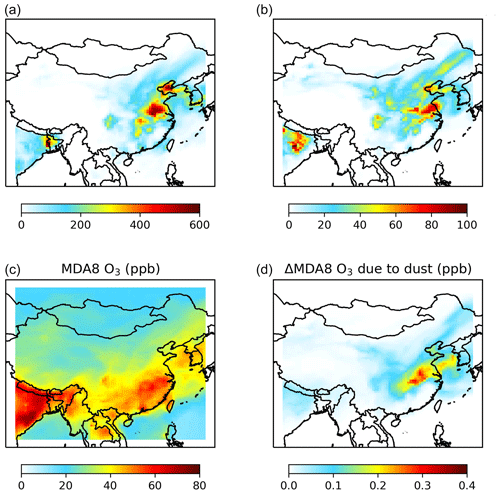
Figure 8Modeled weekly mean mixing ratios of nighttime maximum ClNO2 (a, b) and maximum daily 8 h average (MDA8) ozone (c, d) in surface air over China during 2–7 May 2017. The left panels (a, c) show simulated mixing ratios in our standard case in which φ(ClNO2) is assumed to be 0 for N2O5 uptake onto dust aerosol. The right panels (b, d) show impacts of ClNO2 formation due to N2O5 uptake onto dust, calculated as the difference between the standard case and the case in which φ(ClNO2) is assumed to be 0.1 for N2O5 uptake onto dust.
Figure 8 also shows the effect of ClNO2 formation due to heterogeneous reaction of N2O5 with dust aerosol on the daily maximum 8 h average (MDA8) ozone mixing ratios in the surface air over China during the same period. MDA8 ozone mixing ratios are increased by up to 0.32 ppbv after considering mineral dust as an additional source of ClNO2. Our simulation assumes a low value of φ(ClNO2) in our measured range (<0.05 to ∼0.77) and is conducted in summer when it is more difficult for ClNO2 to accumulate due to short nights (compared to winter and spring with long nights). We expect that its impacts on ClNO2 and ozone could be larger for dust events in winter and spring.
It has been widely recognized that nitryl chloride (ClNO2), produced by heterogeneous reaction of N2O5 with chloride-containing aerosols, could significantly affect atmospheric oxidation capacity. However, heterogeneous formation of tropospheric ClNO2 in inland regions in China has not been well elucidated. In this work, we investigated ClNO2 formation in heterogeneous reaction of N2O5 with eight saline mineral dust samples collected from different regions in China as a function of RH (18 %–75 %). Significant production of ClNO2 was observed for some of the saline mineral dust samples examined, and ClNO2 yields, φ(ClNO2), were determined to span from <0.05 to 0.77, depending on chemical compositions of saline mineral dust samples and RH. In general a positive dependence of φ(ClNO2) on mass fractions of particulate chloride was observed at each RH level, but higher particulate chloride content did not always result in higher φ(ClNO2). On the other hand, an increase in RH could increase, reduce or have no significant impacts on φ(ClNO2), revealing the complex mechanisms which drive heterogeneous uptake of N2O5 onto saline mineral dust.
Two widely used parameterizations (Bertram and Thornton, 2009; Yu et al., 2020) were used to estimate φ(ClNO2) at 75 % RH for the eight saline mineral dust samples we investigated. Both parameterizations were found to significantly overestimate the measured φ(ClNO2), and we suggested that the discrepancies between measured and predicted φ(ClNO2) could be due to incomplete dissolution of particulate chloride, heterogeneous reaction of N2O5 with insoluble minerals, and/or inter- and intra-particle heterogeneity of saline mineral dust particles.
Assuming a φ(ClNO2) value of 0.1 for heterogeneous reaction of N2O5 with mineral dust, we use GEOS-Chem to assess the impact of this reaction on tropospheric ClNO2 and O3 in China during a severe dust event which occurred during 2–7 May 2017. It is found that after taking into account ClNO2 production due to N2O5 uptake onto mineral dust aerosol, weekly mean nighttime maximum ClNO2 mixing ratios could be increased by up to 85 pptv during this period and the daily maximum 8 h average O3 mixing ratios were increased by up to 0.32 ppbv.
In summary, our work shows that heterogeneous reaction of N2O5 with saline mineral dust can be an important source for tropospheric ClNO2 in inland China. This reaction may also be important for tropospheric ClNO2 production in many other regions across the world, as the occurrence of saline mineral dust aerosols has been reported in various locations, such as Iran (Gholampour et al., 2015), the United States (Blank et al., 1999; Pratt et al., 2010; Jordan et al., 2015; Frie et al., 2017) and Argentina (Bucher and Stein, 2016). Currently our limited knowledge precludes quantitative prediction of heterogeneous ClNO2 production from saline mineral dust, and further investigation is thus warranted.
To calibrate CIMS measurements of N2O5, a mixed flow containing N2O5, which was produced via O3 oxidation of NO2, was sampled into the CIMS instrument, and N2O5 was quantified using the normalized intensities of I(N2O5)− clusters, f(N2O5), defined as the ratio of the signal intensity (cps) of I(N2O5)− to that of the total reagent ions, i.e., I− and I(H2O)−. N2O5 concentrations in the mixed flow were quantified using cavity-enhanced absorption spectroscopy (CEAS) (H. Wang et al., 2017), with a detection limit of 2.7 pptv in 5 s and an uncertainty of ∼25 %. RH of the mixed flow was varied during the calibration in order to determine the CIMS sensitivity for N2O5 at different RH levels, and the results are displayed in Fig. A1. The sensitivity for N2O5 first increased with RH, reaching the maximum value at ∼40 % RH, and then decreased with further increases in RH.
To calibrate CIMS measurements of ClNO2, a nitrogen flow (6 mL min−1) containing 10 ppmv of Cl2 was passed over a slurry containing NaNO2 and NaCl to produce ClNO2 (Thaler et al., 2011), and NaCl was included in the slurry in order to minimize the formation of NO2 as a byproduct. The mixed flow containing ClNO2 was then conditioned to a given RH and sampled into the CIMS instrument; similarly to N2O5, ClNO2 was quantified using the normalized intensities of I(ClNO2)− clusters, f(ClNO2), defined as the ratio of the signal intensity (cps) of I(ClNO2)− to that of the total reagent ions. To quantify ClNO2, the mixed flow was delivered directly into a cavity attenuated phase shift spectroscopy (CAPS) instrument (Model N500, Teledyne API) to measure background NO2 concentrations; after that, the mixed flow was delivered through a thermal dissociation model at 365 ∘C to fully decompose ClNO2 to NO2, and the total NO2 concentrations were then determined using CAPS. The differences in the measured NO2 concentrations with and without thermal dissociation were equal to ClNO2 concentrations. The CAPS instrument had a detection limit of 0.2 ppbv in 1 min for NO2 and an uncertainty of ∼10 %. As shown in Fig. A1, the sensitivity for ClNO2 increased with RH up to 40 % and showed little variation with further increases in RH.
Data used in this paper can be found in the main text or Supplement. The GEOS-Chem model is available at the GEOS-Chem repository (http://www.geos-chem.org, GEOS-Chem, 2020).
The supplement related to this article is available online at: https://doi.org/10.5194/acp-22-1845-2022-supplement.
HaW contributed to investigation, formal analysis, writing – original draft, and writing – review and editing; CP contributed to investigation, formal analysis, writing – original draft, and writing – review and editing; XuW contributed to investigation, formal analysis, writing – original draft, and writing – review and editing; SL, HoW, SF and XiW provided resources; KL provided resources and supervision; GG, XJ and XC contributed to investigation; JC provided supervision; MT provided resources and supervision and contributed to conceptualization, formal analysis, writing – original draft, and writing – review and editing.
The contact author has declared that neither they nor their co-authors have any competing interests.
Publisher's note: Copernicus Publications remains neutral with regard to jurisdictional claims in published maps and institutional affiliations.
This work was funded by the National Natural Science Foundation of China (41907185, 42022050 and 91744204), Ministry of Science and Technology of China (2018YFC0213901), Guangdong Basic and Applied Basic Research Fund Committee (2020B1515130003), National State Environmental Protection Key Laboratory of Formation and Prevention of Urban Air Pollution Complex (CX2020080094 and CX2020080578), Guangdong Foundation for Program of Science and Technology Research (2019B121205006 and 2020B1212060053), Guangdong Science and Technology Department (2017GC010501) and CAS Pioneer Hundred Talents program.
This paper was edited by Sachin S. Gunthe and reviewed by two anonymous referees.
Abuduwailli, J., Gabchenko, M. V., and Xu, J.: Eolian transport of salts – A case study in the area of Lake Ebinur (Xinjiang, Northwest China), J. Arid Environ., 72, 1843–1852, 2008.
Ahern, A., Goldberger, L., Jahl, L., Thornton, J., and Sullivan, R. C.: Production of N2O5 and ClNO2 through nocturnal processing of biomass-burning aerosol, Environ. Sci. Technol., 52, 550–559, 2017.
Atkinson, R. and Arey, J.: Atmospheric degradation of volatile organic compounds, Chem. Rev., 103, 4605–4638, 2003.
Atkinson, R., Baulch, D. L., Cox, R. A., Crowley, J. N., Hampson, R. F., Hynes, R. G., Jenkin, M. E., Rossi, M. J., Troe, J., and IUPAC Subcommittee: Evaluated kinetic and photochemical data for atmospheric chemistry: Volume II – gas phase reactions of organic species, Atmos. Chem. Phys., 6, 3625–4055, https://doi.org/10.5194/acp-6-3625-2006, 2006.
Bannan, T. J., Booth, A. M., Bacak, A., Muller, J. B. A., Leather, K. E., Le Breton, M., Jones, B., Young, D., Coe, H., Allan, J., Visser, S., Slowik, J. G., Furger, M., Prevot, A. S. H., Lee, J., Dunmore, R. E., Hopkins, J. R., Hamilton, J. F., Lewis, A. C., Whalley, L. K., Sharp, T., Stone, D., Heard, D. E., Fleming, Z. L., Leigh, R., Shallcross, D. E., and Percival, C. J.: The first UK measurements of nitryl chloride using a chemical ionization mass spectrometer in central London in the summer of 2012, and an investigation of the role of Cl atom oxidation, J. Geophys. Res.-Atmos., 120, 5638–5657, 2015.
Bannan, T. J., Khan, M. A. H., Le Breton, M., Priestley, M., Worrall, S. D., Bacak, A., Marsden, N. A., Lowe, D., Pitt, J., Shallcross, D. E., and Percival, C. J.: A Large Source of Atomic Chlorine From ClNO2 Photolysis at a UK Landfill Site, Geophys. Res. Lett., 46, 8508–8516, 2019.
Bertram, T. H. and Thornton, J. A.: Toward a general parameterization of N2O5 reactivity on aqueous particles: the competing effects of particle liquid water, nitrate and chloride, Atmos. Chem. Phys., 9, 8351–8363, https://doi.org/10.5194/acp-9-8351-2009, 2009.
Blank, R. R., Young, J. A., and Allen, F. L.: Aeolian dust in a saline playa environment, Nevada, USA, J. Arid Environ., 41, 365–381, 1999.
Bucher, E. H. and Stein, A. F.: Large Salt Dust Storms Follow a 30-Year Rainfall Cycle in the Mar Chiquita Lake (Cordoba, Argentina), Plos One, 11, e0156672, https://doi.org/10.1371/journal.pone.0156672, 2016.
Crowley, J. N., Ammann, M., Cox, R. A., Hynes, R. G., Jenkin, M. E., Mellouki, A., Rossi, M. J., Troe, J., and Wallington, T. J.: Evaluated kinetic and photochemical data for atmospheric chemistry: Volume V – heterogeneous reactions on solid substrates, Atmos. Chem. Phys., 10, 9059–9223, https://doi.org/10.5194/acp-10-9059-2010, 2010.
Eger, P. G., Friedrich, N., Schuladen, J., Shenolikar, J., Fischer, H., Tadic, I., Harder, H., Martinez, M., Rohloff, R., Tauer, S., Drewnick, F., Fachinger, F., Brooks, J., Darbyshire, E., Sciare, J., Pikridas, M., Lelieveld, J., and Crowley, J. N.: Shipborne measurements of ClNO2 in the Mediterranean Sea and around the Arabian Peninsula during summer, Atmos. Chem. Phys., 19, 12121–12140, https://doi.org/10.5194/acp-19-12121-2019, 2019.
Faxon, C. B., Bean, J. K., and Hildebrandt Ruiz, L.: Inland Concentrations of Cl-2 and ClNO2 in Southeast Texas Suggest Chlorine Chemistry Significantly Contributes to Atmospheric Reactivity, Atmosphere, 6, 1487–1506, 2015.
Fountoukis, C. and Nenes, A.: ISORROPIA II: a computationally efficient thermodynamic equilibrium model for K+–Ca2+–Mg2+––Na+–––Cl−–H2O aerosols, Atmos. Chem. Phys., 7, 4639–4659, https://doi.org/10.5194/acp-7-4639-2007, 2007.
Frie, A. L., Dingle, J. H., Ying, S. C., and Bahreini, R.: The Effect of a Receding Saline Lake (The Salton Sea) on Airborne Particulate Matter Composition, Environ. Sci. Technol., 51, 8283–8292, 2017.
Fu, X., Wang, T., Wang, S., Zhang, L., Cai, S., Xing, J., and Hao, J.: Anthropogenic Emissions of Hydrogen Chloride and Fine Particulate Chloride in China, Environ. Sci. Technol., 52, 1644–1654, 2018.
Gaston, C. J.: Re-examining Dust Chemical Aging and Its Impacts on Earth's Climate, Accounts Chem. Res., 53, 1005–1013, 2020.
Gaston, C. J., Pratt, K. A., Suski, K. J., May, N. W., Gill, T. E., and Prather, K. A.: Laboratory Studies of the Cloud Droplet Activation Properties and Corresponding Chemistry of Saline Playa Dust, Environ. Sci. Technol., 51, 1348–1356, 2017.
GEOS-Chem [code]: http://www.geos-chem.org, last access: 20 December 2021.
Gholampour, A., Nabizadeh, R., Hassanvand, M. S., Taghipour, H., Nazmara, S., and Mahvi, A. H.: Characterization of saline dust emission resulted from Urmia Lake drying, J. Environ. Health Sci., 13, 82, https://doi.org/10.1186/s40201-015-0238-3, 2015.
Gillette, D., Stensland, G., Williams, A., Barnard, W., Gatz, D., Sinclair, P., and Johnson, T.: Emissions of alkaline elements calcium, magnesium, potassium, and sodium from open sources in the contiguous United States, Global Biogeochem. Cy., 6, 437–457, 1992.
Gu, W., Li, Y., Zhu, J., Jia, X., Lin, Q., Zhang, G., Ding, X., Song, W., Bi, X., Wang, X., and Tang, M.: Investigation of water adsorption and hygroscopicity of atmospherically relevant particles using a commercial vapor sorption analyzer, Atmos. Meas. Tech., 10, 3821–3832, https://doi.org/10.5194/amt-10-3821-2017, 2017.
Guo, L., Gu, W., Peng, C., Wang, W., Li, Y. J., Zong, T., Tang, Y., Wu, Z., Lin, Q., Ge, M., Zhang, G., Hu, M., Bi, X., Wang, X., and Tang, M.: A comprehensive study of hygroscopic properties of calcium- and magnesium-containing salts: implication for hygroscopicity of mineral dust and sea salt aerosols, Atmos. Chem. Phys., 19, 2115–2133, https://doi.org/10.5194/acp-19-2115-2019, 2019.
Jia, X., Gu, W., Peng, C., Li, R., Chen, L., Wang, H., Wang, H., Wang, X., and Tang, M.: Heterogeneous reaction of CaCO3 with NO2 at different relative humidities: Kinetics, mechanisms, and impacts on aerosol hygroscopicity, J. Geophys. Res.-Atmos., 126, e2021JD034826, https://doi.org/10.1029/2021JD034826, 2021.
Jordan, C. E., Pszenny, A. A. P., Keene, W. C., Cooper, O. R., Deegan, B., Maben, J., Routhier, M., Sander, R., and Young, A. H.: Origins of aerosol chlorine during winter over north central Colorado, USA, J. Geophys. Res.-Atmos., 120, 678–694, 2015.
Karagulian, F., Santschi, C., and Rossi, M. J.: The heterogeneous chemical kinetics of N2O5 on CaCO3 and other atmospheric mineral dust surrogates, Atmos. Chem. Phys., 6, 1373–1388, https://doi.org/10.5194/acp-6-1373-2006, 2006.
Kercher, J. P., Riedel, T. P., and Thornton, J. A.: Chlorine activation by N2O5: simultaneous, in situ detection of ClNO2 and N2O5 by chemical ionization mass spectrometry, Atmos. Meas. Tech., 2, 193–204, https://doi.org/10.5194/amt-2-193-2009, 2009.
Le Breton, M., Hallquist, Å. M., Pathak, R. K., Simpson, D., Wang, Y., Johansson, J., Zheng, J., Yang, Y., Shang, D., Wang, H., Liu, Q., Chan, C., Wang, T., Bannan, T. J., Priestley, M., Percival, C. J., Shallcross, D. E., Lu, K., Guo, S., Hu, M., and Hallquist, M.: Chlorine oxidation of VOCs at a semi-rural site in Beijing: significant chlorine liberation from ClNO2 and subsequent gas- and particle-phase Cl–VOC production, Atmos. Chem. Phys., 18, 13013–13030, https://doi.org/10.5194/acp-18-13013-2018, 2018.
Li, R., Jia, X., Wang, F., Ren, Y., Wang, X., Zhang, H., Li, G., Wang, X., and Tang, M.: Heterogeneous reaction of NO2 with hematite, goethite and magnetite: Implications for nitrate formation and iron solubility enhancement, Chemosphere, 242, 125273–125273, 2020.
Lu, K., Guo, S., Tan, Z., Wang, H., Shang, D., Liu, Y., Li, X., Wu, Z., Hu, M., and Zhang, Y.: Exploring atmospheric free-radical chemistry in China: the self-cleansing capacity and the formation of secondary air pollution, Natl. Sci. Rev., 6, 579–594, 2019.
McDuffie, E. E., Fibiger, D. L., Dube, W. P., Hilfiker, F. L., Lee, B. H., Jaegle, L., Guo, H., Weber, R. J., Reeves, J. M., Weinheimer, A. J., Schroder, J. C., Campuzano-Jost, P., Jimenez, J. L., Dibb, J. E., Veres, P., Ebben, C., Sparks, T. L., Wooldridge, P. J., Cohen, R. C., Campos, T., Hall, S. R., Ullmann, K., Roberts, J. M., Thornton, J. A., and Brown, S. S.: ClNO2 Yields From Aircraft Measurements During the 2015 WINTER Campaign and Critical Evaluation of the Current Parameterization, J. Geophys. Res.-Atmos., 123, 12994–13015, 2018a.
McDuffie, E. E., Fibiger, D. L., Dube, W. P., Lopez-Hilfiker, F., Lee, B. H., Thornton, J. A., Shah, V., Jaegle, L., Guo, H., Weber, R. J., Reeves, J. M., Weinheimer, A. J., Schroder, J. C., Campuzano-Jost, P., Jimenez, J. L., Dibb, J. E., Veres, P., Ebben, C., Sparks, T. L., Wooldridge, P. J., Cohen, R. C., Hornbrook, R. S., Apel, E. C., Campos, T., Hall, S. R., Ullmann, K., and Brown, S. S.: Heterogeneous N2O5 Uptake During Winter: Aircraft Measurements During the 2015 WINTER Campaign and Critical Evaluation of Current Parameterizations, J. Geophys. Res.-Atmos., 123, 4345–4372, 2018b.
McNamara, S. M., Kolesar, K. R., Wang, S., Kirpes, R. M., May, N. W., Gunsch, M. J., Cook, R. D., Fuentes, J. D., Hornbrook, R. S., Apel, E. C., China, S., Laskin, A., and Pratt, K. A.: Observation of Road Salt Aerosol Driving Inland Wintertime Atmospheric Chlorine Chemistry, ACS Central Science, 6, 684–694, 2020.
Mielke, L. H., Furgeson, A., and Osthoff, H. D.: Observation of CINO2 in a Mid-Continental Urban Environment, Environ. Sci. Technol., 45, 8889–8896, 2011.
Mielke, L. H., Furgeson, A., Odame-Ankrah, C. A., and Osthoff, H. D.: Ubiquity of ClNO2 in the urban boundary layer of Calgary, Alberta, Canada, Can. J. Chem., 94, 414–423, 2016.
Mitroo, D., Gill, T. E., Haas, S., Pratt, K. A., and Gaston, C. J.: ClNO2 Production from N2O5 Uptake on Saline Playa Dusts: New Insights into Potential Inland Sources of ClNO2, Environ. Sci. Technol., 53, 7442–7452, 2019.
Osthoff, H. D., Roberts, J. M., Ravishankara, A. R., Williams, E. J., Lerner, B. M., Sommariva, R., Bates, T. S., Coffman, D., Quinn, P. K., Dibb, J. E., Stark, H., Burkholder, J. B., Talukdar, R. K., Meagher, J., Fehsenfeld, F. C., and Brown, S. S.: High levels of nitryl chloride in the polluted subtropical marine boundary layer, Nat. Geosci., 1, 324–328, 2008.
Osthoff, H. D., Odame-Ankrah, C. A., Taha, Y. M., Tokarek, T. W., Schiller, C. L., Haga, D., Jones, K., and Vingarzan, R.: Low levels of nitryl chloride at ground level: nocturnal nitrogen oxides in the Lower Fraser Valley of British Columbia, Atmos. Chem. Phys., 18, 6293–6315, https://doi.org/10.5194/acp-18-6293-2018, 2018.
Phillips, G. J., Tang, M. J., Thieser, J., Brickwedde, B., Schuster, G., Bohn, B., Lelieveld, J., and Crowley, J. N.: Significant concentrations of nitryl chloride observed in rural continental Europe associated with the influence of sea salt chloride and anthropogenic emissions, Geophys. Res. Lett., 39, L10811, https://doi.org/10.1029/2012gl051912, 2012.
Pratt, K. A., Twohy, C. H., Murphy, S. M., Moffet, R. C., Heymsfield, A. J., Gaston, C. J., DeMott, P. J., Field, P. R., Henn, T. R., Rogers, D. C., Gilles, M. K., Seinfeld, J. H., and Prather, K. A.: Observation of playa salts as nuclei in orographic wave clouds, J. Geophys. Res.-Atmos., 115, D15301, https://doi.org/10.1029/2009JD013606, 2010.
Ridley, D. A., Heald, C. L., Pierce, J. R., and Evans, M. J.: Toward resolution-independent dust emissions in global models: Impacts on the seasonal and spatial distribution of dust, Geophys. Res. Lett., 40, 2873–2877, 2013.
Riedel, T. P., Bertram, T. H., Crisp, T. A., Williams, E. J., Lerner, B. M., Vlasenko, A., Li, S.-M., Gilman, J., de Gouw, J., Bon, D. M., Wagner, N. L., Brown, S. S., and Thornton, J. A.: Nitryl Chloride and Molecular Chlorine in the Coastal Marine Boundary Layer, Environ. Sci. Technol., 46, 10463–10470, 2012.
Riedel, T. P., Wagner, N. L., Dube, W. P., Middlebrook, A. M., Young, C. J., Ozturk, F., Bahreini, R., VandenBoer, T. C., Wolfe, D. E., Williams, E. J., Roberts, J. M., Brown, S. S., and Thornton, J. A.: Chlorine activation within urban or power plant plumes: Vertically resolved ClNO2 and Cl-2 measurements from a tall tower in a polluted continental setting, J. Geophys. Res.-Atmos., 118, 8702–8715, 2013.
Riedel, T. P., Wolfe, G. M., Danas, K. T., Gilman, J. B., Kuster, W. C., Bon, D. M., Vlasenko, A., Li, S.-M., Williams, E. J., Lerner, B. M., Veres, P. R., Roberts, J. M., Holloway, J. S., Lefer, B., Brown, S. S., and Thornton, J. A.: An MCM modeling study of nitryl chloride (ClNO2) impacts on oxidation, ozone production and nitrogen oxide partitioning in polluted continental outflow, Atmos. Chem. Phys., 14, 3789–3800, https://doi.org/10.5194/acp-14-3789-2014, 2014.
Royer, H. M., Mitroo, D., Hayes, S. M., Haas, S. M., Pratt, K. A., Blackwelder, P. L., Gill, T. E., and Gaston, C. J.: The Role of Hydrates, Competing Chemical Constituents, and Surface Composition on ClNO2 Formation, Environ. Sci. Technol., 55, 2869–2877, https://doi.org/10.1021/acs.est.0c06067, 2021.
Ryder, O. S., Ault, A. P., Cahill, J. F., Guasco, T. L., Riedel, T. P., Cuadra-Rodriguez, L. A., Gaston, C. J., Fitzgerald, E., Lee, C., Prather, K. A., and Bertram, T. H.: On the Role of Particle Inorganic Mixing State in the Reactive Uptake of N2O5 to Ambient Aerosol Particles, Environ. Sci. Technol., 48, 1618–1627, 2014.
Saiz-Lopez, A. and von Glasow, R.: Reactive halogen chemistry in the troposphere, Chem. Soc. Rev., 41, 6448–6472, 2012.
Sarwar, G., Simon, H., Xing, J., and Mathur, R.: Importance of tropospheric ClNO2 chemistry across the Northern Hemisphere, Geophys. Res. Lett., 41, 4050–4058, 2014.
Seisel, S., Börensen, C., Vogt, R., and Zellner, R.: Kinetics and mechanism of the uptake of N2O5 on mineral dust at 298 K, Atmos. Chem. Phys., 5, 3423–3432, https://doi.org/10.5194/acp-5-3423-2005, 2005.
Simon, H., Kimura, Y., McGaughey, G., Allen, D. T., Brown, S. S., Osthoff, H. D., Roberts, J. M., Byun, D., and Lee, D.: Modeling the impact of ClNO2 on ozone formation in the Houston area, J. Geophys. Res.-Atmos., 114, D00F03, https://doi.org/10.1029/2008jd010732, 2009.
Simpson, W. R., Brown, S. S., Saiz-Lopez, A., Thornton, J. A., and von Glasow, R.: Tropospheric Halogen Chemistry: Sources, Cycling, and Impacts, Chem. Rev., 115, 4035–4062, 2015.
Tang, M., Huang, X., Lu, K., Ge, M., Li, Y., Cheng, P., Zhu, T., Ding, A., Zhang, Y., Gligorovski, S., Song, W., Ding, X., Bi, X., and Wang, X.: Heterogeneous reactions of mineral dust aerosol: implications for tropospheric oxidation capacity, Atmos. Chem. Phys., 17, 11727–11777, https://doi.org/10.5194/acp-17-11727-2017, 2017.
Tang, M., Zhang, H., Gu, W., Gao, J., Jian, X., Shi, G., Zhu, B., Xie, L., Guo, L., and Gao, X.: Hygroscopic properties of saline mineral dust from different regions in China: geographical variations, compositional dependence and atmospheric implications, J. Geophys. Res.-Atmos., 124, 10844–10857, 2019.
Tang, M. J., Thieser, J., Schuster, G., and Crowley, J. N.: Kinetics and mechanism of the heterogeneous reaction of N2O5 with mineral dust particles, Phys. Chem. Chem. Phys., 14, 8551–8561, 2012.
Thaler, R. D., Mielke, L. H., and Osthoff, H. D.: Quantification of Nitryl Chloride at Part Per Trillion Mixing Ratios by Thermal Dissociation Cavity Ring-Down Spectroscopy, Anal. Chem., 83, 2761–2766, 2011.
Tham, Y. J., Yan, C., Xue, L., Zha, Q., Wang, X., and Wang, T.: Presence of high nitryl chloride in Asian coastal environment and its impact on atmospheric photochemistry, Chinese Sci. Bull., 59, 356–359, 2014.
Tham, Y. J., Wang, Z., Li, Q., Yun, H., Wang, W., Wang, X., Xue, L., Lu, K., Ma, N., Bohn, B., Li, X., Kecorius, S., Größ, J., Shao, M., Wiedensohler, A., Zhang, Y., and Wang, T.: Significant concentrations of nitryl chloride sustained in the morning: investigations of the causes and impacts on ozone production in a polluted region of northern China, Atmos. Chem. Phys., 16, 14959–14977, https://doi.org/10.5194/acp-16-14959-2016, 2016.
Tham, Y. J., Wang, Z., Li, Q., Wang, W., Wang, X., Lu, K., Ma, N., Yan, C., Kecorius, S., Wiedensohler, A., Zhang, Y., and Wang, T.: Heterogeneous N2O5 uptake coefficient and production yield of ClNO2 in polluted northern China: roles of aerosol water content and chemical composition, Atmos. Chem. Phys., 18, 13155–13171, https://doi.org/10.5194/acp-18-13155-2018, 2018.
Thornton, J. A., Kercher, J. P., Riedel, T. P., Wagner, N. L., Cozic, J., Holloway, J. S., Dube, W. P., Wolfe, G. M., Quinn, P. K., Middlebrook, A. M., Alexander, B., and Brown, S. S.: A large atomic chlorine source inferred from mid-continental reactive nitrogen chemistry, Nature, 464, 271–274, 2010.
Wang, H., Chen, J., and Lu, K.: Development of a portable cavity-enhanced absorption spectrometer for the measurement of ambient NO3 and N2O5: experimental setup, lab characterizations, and field applications in a polluted urban environment, Atmos. Meas. Tech., 10, 1465–1479, https://doi.org/10.5194/amt-10-1465-2017, 2017.
Wang, H., Lu, K., Guo, S., Wu, Z., Shang, D., Tan, Z., Wang, Y., Le Breton, M., Lou, S., Tang, M., Wu, Y., Zhu, W., Zheng, J., Zeng, L., Hallquist, M., Hu, M., and Zhang, Y.: Efficient N2O5 uptake and NO3 oxidation in the outflow of urban Beijing, Atmos. Chem. Phys., 18, 9705–9721, https://doi.org/10.5194/acp-18-9705-2018, 2018.
Wang, H., Tang, M., Tan, Z., Peng, C., and Lu, K.: Atmospheric Chemistry of Nitryl Chloride, Prog. Chem., 32, 1535–1546, 2020.
Wang, T., Tham, Y. J., Xue, L., Li, Q., Zha, Q., Wang, Z., Poon, S. C. N., Dube, W. P., Blake, D. R., Louie, P. K. K., Luk, C. W. Y., Tsui, W., and Brown, S. S.: Observations of nitryl chloride and modeling its source and effect on ozone in the planetary boundary layer of southern China, J. Geophys. Res.-Atmos., 121, 2476–2489, 2016.
Wang, X., Hua, T., Zhang, C., Lang, L., and Wang, H.: Aeolian salts in Gobi deserts of the western region of Inner Mongolia: Gone with the dust aerosols, Atmos. Res., 118, 1–9, 2012.
Wang, X., Wang, H., Xue, L., Wang, T., Wang, L., Gu, R., Wang, W., Tham, Y. J., Wang, Z., Yang, L., Chen, J., and Wang, W.: Observations of N2O5 and ClNO2 at a polluted urban surface site in North China: High N2O5 uptake coefficients and low ClNO2 product yields, Atmos. Environ., 156, 125–134, 2017.
Wang, X., Jacob, D. J., Eastham, S. D., Sulprizio, M. P., Zhu, L., Chen, Q., Alexander, B., Sherwen, T., Evans, M. J., Lee, B. H., Haskins, J. D., Lopez-Hilfiker, F. D., Thornton, J. A., Huey, G. L., and Liao, H.: The role of chlorine in global tropospheric chemistry, Atmos. Chem. Phys., 19, 3981–4003, https://doi.org/10.5194/acp-19-3981-2019, 2019.
Wang, X., Jacob, D. J., Fu, X., Wang, T., Le Breton, M., Hallquist, M., Liu, Z., McDuffie, E. E., and Liao, H.: Effects of Anthropogenic Chlorine on PM2.5 and Ozone Air Quality in China, Environ. Sci. Technol., 54, 9908–9916, 2020.
Wang, X., Jacob, D. J., Downs, W., Zhai, S., Zhu, L., Shah, V., Holmes, C. D., Sherwen, T., Alexander, B., Evans, M. J., Eastham, S. D., Neuman, J. A., Veres, P. R., Koenig, T. K., Volkamer, R., Huey, L. G., Bannan, T. J., Percival, C. J., Lee, B. H., and Thornton, J. A.: Global tropospheric halogen (Cl, Br, I) chemistry and its impact on oxidants, Atmos. Chem. Phys., 21, 13973–13996, https://doi.org/10.5194/acp-21-13973-2021, 2021.
Wang, Z., Wang, W., Tham, Y. J., Li, Q., Wang, H., Wen, L., Wang, X., and Wang, T.: Fast heterogeneous N2O5 uptake and ClNO2 production in power plant and industrial plumes observed in the nocturnal residual layer over the North China Plain, Atmos. Chem. Phys., 17, 12361–12378, https://doi.org/10.5194/acp-17-12361-2017, 2017.
Wu, C., Zhang, S., Wang, G., Lv, S., Li, D., Liu, L., Li, J., Liu, S., Du, W., Meng, J., Qiao, L., Zhou, M., Huang, C., and Wang, H.: Efficient Heterogeneous Formation of Ammonium Nitrate on the Saline Mineral Particle Surface in the Atmosphere of East Asia during Dust Storm Periods, Environ. Sci. Technol., 54, 15622–15630, 2020.
Young, C. J., Washenfelder, R. A., Edwards, P. M., Parrish, D. D., Gilman, J. B., Kuster, W. C., Mielke, L. H., Osthoff, H. D., Tsai, C., Pikelnaya, O., Stutz, J., Veres, P. R., Roberts, J. M., Griffith, S., Dusanter, S., Stevens, P. S., Flynn, J., Grossberg, N., Lefer, B., Holloway, J. S., Peischl, J., Ryerson, T. B., Atlas, E. L., Blake, D. R., and Brown, S. S.: Chlorine as a primary radical: evaluation of methods to understand its role in initiation of oxidative cycles, Atmos. Chem. Phys., 14, 3427–3440, https://doi.org/10.5194/acp-14-3427-2014, 2014.
Yu, C., Wang, Z., Xia, M., Fu, X., Wang, W., Tham, Y. J., Chen, T., Zheng, P., Li, H., Shan, Y., Wang, X., Xue, L., Zhou, Y., Yue, D., Ou, Y., Gao, J., Lu, K., Brown, S. S., Zhang, Y., and Wang, T.: Heterogeneous N2O5 reactions on atmospheric aerosols at four Chinese sites: improving model representation of uptake parameters, Atmos. Chem. Phys., 20, 4367–4378, https://doi.org/10.5194/acp-20-4367-2020, 2020.
Zhang, H., Gu, W., Li, Y. J., and Tang, M.: Hygroscopic properties of sodium and potassium salts as related to saline mineral dusts and sea salt aerosols, J. Environ. Sci., 95, 65–72, 2020.
Zhang, X., Zhuang, G., Yuan, H., Rahn, K. A., Wang, Z., and An, Z.: Aerosol Particles from Dried Salt-Lakes and Saline Soils Carried on Dust Storms over Beijing, Terr. Atmos. Ocean. Sci., 20, 619–628, 2009.
Zhang, X.-X., Sharratt, B., Liu, L.-Y., Wang, Z.-F., Pan, X.-L., Lei, J.-Q., Wu, S.-X., Huang, S.-Y., Guo, Y.-H., Li, J., Tang, X., Yang, T., Tian, Y., Chen, X.-S., Hao, J.-Q., Zheng, H.-T., Yang, Y.-Y., and Lyu, Y.-L.: East Asian dust storm in May 2017: observations, modelling, and its influence on the Asia-Pacific region, Atmos. Chem. Phys., 18, 8353–8371, https://doi.org/10.5194/acp-18-8353-2018, 2018.
Zheng, B., Tong, D., Li, M., Liu, F., Hong, C., Geng, G., Li, H., Li, X., Peng, L., Qi, J., Yan, L., Zhang, Y., Zhao, H., Zheng, Y., He, K., and Zhang, Q.: Trends in China's anthropogenic emissions since 2010 as the consequence of clean air actions, Atmos. Chem. Phys., 18, 14095–14111, https://doi.org/10.5194/acp-18-14095-2018, 2018.