the Creative Commons Attribution 4.0 License.
the Creative Commons Attribution 4.0 License.
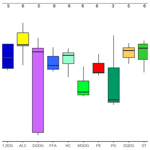
Concerted measurements of lipids in seawater and on submicrometer aerosol particles at the Cabo Verde islands: biogenic sources, selective transfer and high enrichments
Nadja Triesch
Manuela van Pinxteren
Sanja Frka
Christian Stolle
Tobias Spranger
Erik Hans Hoffmann
Xianda Gong
Heike Wex
Detlef Schulz-Bull
Blaženka Gašparović
In the marine environment, measurements of lipids as representative species within different lipid classes have been performed to characterize their oceanic sources and their transfer from the ocean into the atmosphere to marine aerosol particles. The set of lipid classes includes hydrocarbons (HC); fatty acid methyl esters (ME); free fatty acids (FFA); alcohols (ALC); 1,3-diacylglycerols (1,3 DG); 1,2-diacylglycerols (1,2 DG); monoacylglycerols (MG); wax esters (WE); triacylglycerols (TG); and phospholipids (PP) including phosphatidylglycerols (PG), phosphatidylethanolamine (PE), phosphatidylcholines (PC), as well as glycolipids (GL) which cover sulfoquinovosyldiacylglycerols (SQDG), monogalactosyl-diacylglycerols (MGDG), digalactosyldiacylglycerols (DGDG) and sterols (ST). These introduced lipid classes have been analyzed in the dissolved and particulate fraction of seawater, differentiating between underlying water (ULW) and the sea surface microlayer (SML) on the one hand. On the other hand, they have been examined on ambient submicrometer aerosol particle samples (PM1) which were collected at the Cape Verde Atmospheric Observatory (CVAO) by applying concerted measurements. These different lipids are found in all marine compartments but in different compositions. Along the campaign, certain variabilities are observed for the concentration of dissolved (∑DLULW: 39.8–128.5 µg L−1, ∑DLSML: 55.7–121.5 µg L−1) and particulate (∑PLULW: 36.4–93.5 µg L−1, ∑PLSML: 61.0–118.1 µg L−1) lipids in the seawater of the tropical North Atlantic Ocean. Only slight SML enrichments are observed for the lipids with an enrichment factor EFSML of 1.1–1.4 (DL) and 1.0–1.7 (PL). On PM1 aerosol particles, a total lipid concentration between 75.2–219.5 ng m−3 (averaged: 119.9 ng m−3) is measured. As also bacteria – besides phytoplankton sources – influence the lipid concentrations in seawater and on the aerosol particles, the lipid abundance cannot be exclusively explained by the phytoplankton tracer (chlorophyll a). The concentration and enrichment of lipids in the SML are not related to physicochemical properties which describe the surface activity. On the aerosol particles, an EFaer (the enrichment factor on the submicrometer aerosol particles compared to the SML) between 9×104–7×105 is observed. Regarding the individual lipid groups on the aerosol particles, a statistically significant correlation (R2=0.45, p=0.028) was found between EFaer and lipophilicity (expressed by the KOW value), which was not present for the SML. But simple physicochemical descriptors are overall not sufficient to fully explain the transfer of lipids. As our findings show that additional processes such as formation and degradation influence the ocean–atmosphere transfer of both OM in general and of lipids in particular, they have to be considered in OM transfer models. Moreover, our data suggest that the extent of the enrichment of the lipid class constituents on the aerosol particles might be related to the distribution of the lipid within the bubble–air–water interface. The lipids TG and ALC which are preferably arranged within the bubble interface are transferred to the aerosol particles to the highest extent. Finally, the connection between ice nucleation particles (INPs) in seawater, which are already active at higher temperatures (−10 to −15 ∘C), and the lipid classes PE and FFA suggests that lipids formed in the ocean have the potential to contribute to (biogenic) INP activity when transferred into the atmosphere.
- Article
(1891 KB) - Full-text XML
-
Supplement
(2009 KB) - BibTeX
- EndNote
Lipids are a major biochemical class of organic matter (OM) in seawater along with carbohydrates and proteins. Their ocean concentrations are much lower, yet their surface affinity and enrichment are higher than for the other groups (Burrows et al., 2014). As lipids are rich in carbon and serve as energy storage compounds, they are important components of the cellular metabolisms of species, at least in the ocean (Wakeham et al., 1997). They are distributed throughout the marine environment and are involved in numerous essential biological processes of both the dissolved and particulate OM pool (Arts et al., 2001; Frka et al., 2011). As regards the particulate lipid pool, important sources are phytoplankton cells with a contribution of up to 79 % in biologically productive surface water layers, while the contribution of lipids in phytoplankton cells ranges between ≤1 % and 46 % of dry weight (Frka et al., 2011, and references therein). Marine lipids can be produced by abiotic and biotic processes and play an important role as energy sources in the aquatic ecosystem (Parrish, 2013). As a whole, the analysis of lipids and their classes is a useful method to study the dynamics of the global carbon cycle in the ocean (Yoshimura et al., 2009), since lipid classes can be used as specific markers for the identification of OM sources and biogeochemical cycles in the marine environment (Parrish et al., 1988; Frka et al., 2011). Phosphorus-containing lipids or phospholipids (PP), i.e., phosphatidylglycerols (PG), phosphatidylethanolamine (PE) and phosphatidylcholines (PC), belong to the organic substances associated with living organisms (Derieux et al., 1998) since they are a major component of cell membranes providing the structure and protection of cells (Khozin-Goldberg, 2016). The most common glycolipids (GL) in plankton are mono- and di-galactosyldiacylglycerols (MGDG and DGDG) as well as sulfoquinovosyldiacylglycerol (SQDG) (Guschina and Harwood, 2009; Gašparović et al., 2013). Triacylglycerols (TG) indicate metabolic reserves (Frka et al., 2011) and are reported as storage compounds in phytoplankton (Becker et al., 2018). Wax esters (WE) are a major group of neutral lipids of some zooplankton species (Kattner, 1989). Fatty alcohols (ALC) mainly originate from zooplankton wax esters (Frka et al., 2011). Diacylglycerides (DG), monoacylglycerides (MG) and free fatty acids (FFA) are glyceride degradation products which characterize the degradation level of lipids by means of the lipolysis index (LI) (Parrish et al., 1988; Goutx et al., 2003). By using lipids as biomarkers, estimates of marine OM sinks and sources can be made and previous OM parameterizations, based only on chl a, can be extended. Indeed, chl a is often used as an indicator of marine biological productivity, representing the abundance of the major group of organisms, i.e., the photoautotrophs (Gantt et al., 2011; Rinaldi et al., 2013). To fully describe the biological control of the OM cycle, both autotrophic and heterotrophic organisms must be considered. The information on the abundance of the main OM classes, namely lipids, proteins and carbohydrates, contributing to the marine OM pool and reflecting the OM sources, can therefore be used for advanced modeling approaches to predict OM on marine aerosol particles depending on the chemical composition of marine OM, as was described by Burrows et al. (2014), for example. Further spatiotemporal investigations of the organic classes in different oceanic areas are important for this purpose. The study of lipids is particularly important because their reactivity and physical surface properties contribute to the formation and stabilization of the sea surface microlayer (SML) (Frka et al., 2012, and references therein). The SML represents a chemically distinct film enriched with surface active OM that accumulates at the air–water interface relative to the underlying water (ULW) (Wurl and Holmes, 2008; Wurl et al., 2011; Cunliffe et al., 2013). So, the SML as a natural interface between ocean and atmosphere could play an important role as an OM source for aerosol particles in the marine environment (Engel et al., 2017). The presence of lipids at the air–water interface is the result of their high surface affinity, competitive adsorption and segregation from other OM constituents (Frka et al., 2012).
OM can be transferred from the ocean into the atmosphere by wind-driven processes and bubble bursting, resulting in the formation of primary marine aerosol particles. Within this process, film drops leading to the formation of submicrometer particles are enriched mainly with OM compared to larger jet drops (Wilson et al., 2015). According to most parameterizations, the transfer of OM and the prediction of the OM content on marine aerosol particles are based on chl a seawater concentrations that are used as a broad indicator of biological productivity (Gantt et al., 2011; Rinaldi et al., 2013). However, especially in oligotrophic regions additional parameters besides wind speed and chl a must be taken into consideration for an accurate prediction of OM on marine aerosol particles (van Pinxteren et al., 2017). In a new approach by Burrows et al. (2014) the parameterization and OM prediction for marine aerosol particles is based on important compound classes of OM, e.g., lipids, carbohydrates and proteins, with different physicochemical properties instead of the chl a concentrations of the seawater. This new approach requires information of distinct OM groups in both marine matrices (seawater and aerosol particles) such as the lipid concentrations performed in the present study.
Specific lipid classes such as long-chain fatty acids and cholesterol as constituents of aerosol particles are already regarded as important factors for the activation of aerosol particles to cloud condensation nuclei (CCN) (Barati et al., 2019) or ice nucleation particles (INPs) (Nguyen et al., 2017; DeMott et al., 2018). Cochran et al. (2016b) investigated the fatty acid composition on sub- and supermicrometer sea spray aerosol particles and reported that about 75 % of the submicrometer aerosol particles showed clear signals for the presence of long-chain fatty acids. In contrast, supermicrometer sea spray aerosol particles were dominated (up to 88 %) by oxygen-rich species (Cochran et al., 2016b).
Until now, lipids or individual lipid classes have often been analyzed in only one compartment of the ambient marine environment, i.e., seawater or marine aerosol particles (Gagosian et al., 1982; Simoneit and Mazurek, 1982; Frka et al., 2011; Gašparović et al., 2014). The possible transfer of total lipids (determined as a sum parameter) has so far only been described under controlled conditions in laboratory studies (Rastelli et al., 2017) or for certain lipid classes such as fatty acids, n-alkanes, total hydrocarbons (Marty et al., 1979), or as fatty acids and their derivatives (Cochran et al., 2016b) in freshly emitted sea spray aerosol. Unfortunately, comprehensive studies on marine lipid biogeochemistry and on a possible transfer of certain lipid classes into the atmosphere are missing.
The present work aims at investigating lipids at the Cape Verde Atmospheric Observatory (CVAO) as species representative for different lipid classes in the marine environment of the tropical Atlantic Ocean, to study their abundance, (biogenic) sources and selective transfer into the marine atmosphere. The lipid data set obtained for samples from different marine compartments at the CVAO is discussed with regard to its biological origin and its ice nucleation potential. Finally, the potential transfer of the lipids from seawater to aerosol particles will be investigated by relating the physicochemical properties of the individual lipid classes to their respective observed atmospheric enrichment.
2.1 Study area and sampling sites
As part of the MarParCloud project (Marine biological production, organic aerosol particles and marine clouds: a Process Chain) with a contribution from MARSU (MARine atmospheric Science Unravelled: Analytical and mass spectrometric techniques development and application), a field campaign was performed at the Cape Verde Atmospheric Observatory (CVAO, 16∘51′49′′ N, 24∘52′02′′ E) from 13 September to 13 October 2017 (Triesch et al., 2021; van Pinxteren et al., 2020). The CVAO, a remote marine station in the tropical Atlantic Ocean located on the northeast coast of Sao Vicente island, is described in more detail in Carpenter et al. (2010) and Fomba et al. (2014). The ocean around the Cabo Verde islands belongs to the region “North Atlantic Tropical Gyral Province (NATR)” according to the classification of Longhurst (2007), a region with the lowest surface chlorophyll in the North Atlantic and with a greater annual variability than seasonality. Wilson et al. (2015) reported that high concentrations of marine INPs can occur in the North Atlantic and that comparatively high surface-level marine (INP15) and OC concentrations have been predicted by models in this region around the Cabo Verde islands. A concerted sampling was carried out during the campaign, including PM1 aerosol particles at the CVAO and seawater at the ocean site (∼16∘53′17′′ N, ∼24∘54′25′′ E). The seawater sampling site was located upwind of the CVAO and had a minimal island influence. A map illustrating the seawater sampling station and the CVAO is shown in Fig. S1.
2.1.1 SML and seawater sampling
The SML samples (n=6) were collected using the manual glass-plate technique, a standard SML sampling method whose correct application and specification are described in detail in the “Guide to best practices to study the ocean's surface” by Cunliffe (2014). A glass plate (500×250 mm) with a sampling area of 2500 cm2 was briefly immersed vertically in the seawater and then slowly pulled out. The surface film material adsorbed on the surface of the glass plate was then directly put into pre-cleaned bottles using Teflon wipers. The ULW samples (n=13) were collected from a depth of 1 m and also put in pre-cleaned plastic bottles, which were attached to a telescopic rod. The bottles were opened under water at the intended sampling depth in order to avoid influences by the SML. In addition, 5–6 L of bulk surface water (at a depth between 10 and 50 cm) were collected with a plastic bottle for the pigment analysis (n=11).
2.1.2 Aerosol particles sampling
At the top of a 30 m high sampling tower at the CVAO, PM1 aerosol particles (n=13) were collected using a high-volume Digitel sampler DHA-80 (Walter Riemer Messtechnik, Germany) on 150 mm quartz fiber filters (Munktell, MK 360) at a flow rate of 700 L min−1 in 24 h. The filters used for sampling were preheated at 105 ∘C for 24 h to avoid contamination. The collected filters were stored in aluminum boxes at −20 ∘C up until analyzed.
2.2 Analytics
2.2.1 Seawater samples analytics: lipids, pigments, nutrients, microorganisms, INP activity
For the lipid analysis, the seawater samples (2 L) were passed through a 200 µm stainless steel screen to remove zooplankton and larger particles. Afterwards, they were filtered through pre-combusted (350 ∘C for 5 h) 0.7 µm GF/F filters (Whatman®, Sigma Aldrich). After the filtration step, the internal standard 2-hexadecanone (purity ≥98 %, Sigma Aldrich) (10 µg) was added to each filtrate, while the corresponding filters were stored in tubes at −78 ∘C until analyzed. Dissolved lipids (DL) were immediately extracted from the filtrate with dichloromethane (DCM; dichloromethane for liquid chromatography LiChrosolv®, Merck) in four extraction steps (twice at pH 8 and twice at pH 2). The final extract was concentrated using a rotary evaporator. Particulate lipids (PL) were extracted by a modified one-phase solvent mixture of DCM–methanol–water (Bligh and Dyer, 1959) after the addition of 2-hexadecanone as internal standard. DL and PL classes were further analyzed by Iatroscan thin layer chromatography and flame ionization detection (TLC-FID) (Iatroscan MK-VI, Iatron, Japan) as described in Gašparović et al. (2015, 2017). After the separation on Chromarod-SIII thin layer rods, lipid classes were identified and quantified by external calibration with a standard lipid mixture. The investigated lipid classes included HC, ME, FFA, ALC, 1,3 DG, 1,2 DG, MG, WE, TG, PP (including PG, PE, PC), GL (including SQDG, MGDG, DGDG), ST and PIG. Each sample was measured twice with a relative standard deviation <10 %. Also, blank seawater samples (high-purity water filled in pre-cleaned plastic bottles and handled the same as the seawater samples) were analyzed, and the blank values were always below 15 % of ambient seawater samples. All presented lipid values for the SML and ULW samples were blank-corrected by subtracting the field blank values from the samples.
For the pigment analysis, 5–6 L of bulk water was filtrated through GF/F filters. These were extracted in 5 mL ethanol, and an aliquot (20 µL) was injected into a high-performance liquid chromatograph system (HPLC) with fluorescence detection (Dionex, Sunnyvale, CA. USA). The pigments, including chl a, chl b, fucoxanthin, pheophorbide, pheophytin a/b, zeaxanthin, diadinoxanthin, lutein, chlorophyllide, violaxanthin and β-carotene, were separated under gradient elution using methanol–acetonitrile–water systems as the mobile phase, as described in van Pinxteren et al. (2019). Nutrients covering nitrogen oxides (N2O3, NO2, NO3), phosphates (PO) and silicates (SiO) were measured colorimetrically according to Grasshoff et al. (1999) with a Seal Analytical QuAAtro constant flow analyzer. Microbial cell numbers were counted via flow cytometry after the seawater samples were fixed, flash-frozen in liquid nitrogen and stored at −20 ∘C. For the prokaryotic cells counts, all samples were stained with SYBR Green solution. The counting was performed after the addition of latex beads serving as an internal standard. Further details can be found in Robinson et al. (2019). Small autotrophic cells were counted in a separate measurement after adding red fluorescent latex beads (Polysciences, Eppelheim, Germany). Cells were detected by their signature in a plot of red (FL3) vs. orange (FL2) fluorescence, and red fluorescence vs. side scatter (SSC). This approach allows discrimination between different groups of prokaryotic and eukaryotic autotrophs (Marie et al., 2010), which in our case were size classes defined as Synechococcus-like cells and Nanoeucaryotes. Two droplet freezing devices called LINA (Leipzig Ice Nucleation Array) and INDA (Ice Nucleation Droplet Array) are used at TROPOS to obtain information on the ice nucleation activity as described in more detail by Gong et al. (2020) and references therein.
2.2.2 Aerosol particle samples analytics
The analysis of sodium (Na+) was performed by ion chromatography (ICS3000, Dionex, Sunnyvale, CA, USA) as described in Müller et al. (2010). For the analysis of the lipid classes, 28.27 cm2 of the total PM1 filter area were extracted and measured following the procedure for particulate lipids in seawater (see Sect. 2.2.1). A chromatogram of the TLC-FID measurements of an aerosol particle sample and the standards is shown in Fig. S2. The field blanks (n=5) were prepared using pre-baked quartz fiber filters without an active sampling and treated according to the same procedure as the field samples. The concentrations of the lipid classes were calculated by external calibration. Each sample was measured twice with a relative standard deviation <10 %, and field blanks, which were always below 20 % of the real aerosol particle sample, were subtracted. All presented values are corrected for the field blank.
2.2.3 Lipid ratios
The lipolysis index (LI) is an index describing the lipid degradative state and the biological degradation processes of lipids (Goutx et al., 2003). This concept describes the degradation process of marine acyl-lipids by the concentration ratio of free lipids and metabolites (ALC, FFA, MG, DG) to their precursors (TG, WE, glycolipids as MGDG, DGDG, SQDG and phospholipids as PG, PE, PC) by Eq. (1).
It is obvious that the concentration of precursor lipids and metabolites alone can already influence the LI and that there may be a natural variance between them. For example, metabolites can be quickly absorbed by existing microorganisms, which would lead to a reduction of the concentration and thus influence the LI. However, the LI is reported as a useful measure to characterize the degradation level of biogenic organic material (Goutx et al., 2003). Higher LI values are characteristic for an enhanced OM degradation and metabolite release, while lower LI values indicate that the appearing lipid classes are more fresh or resistant to degradation.
The PE PG ratio can be used to determine the origin of the phospholipids that contribute to the OM pool in seawater (Goutx et al., 1993). Here, PG as the most important compound of the phospholipids of microalgae is an indicator for algae as potential sources, whereas PE is predominantly found in bacterial membranes and thus represents an indicator for bacterial sources (Goutx et al., 1993).
2.2.4 Enrichment factors
The enrichment factor in the SML (EFSML) was calculated by dividing the concentration of the respective analyte in the SML by the concentration of the analyte in the ULW using Eq. (2).
An enrichment in the SML is defined as EFSML>1, a depletion with EFSML<1.
The EFaer is a quantitative metric for the comparison of compounds in the ocean and in the atmosphere. The EFaer concept is mainly applied to closed systems (Quinn et al., 2015, and references therein, Rastelli et al., 2017) since formation or degradation pathways on aerosol particles including biological or photochemical atmospheric reactions and possible transports from other sources than marine sources are excluded from this parameter. Nevertheless, for comparison purposes it is useful to also apply the EFaer for open systems, as in the studies of Russell et al. (2010) or van Pinxteren et al. (2017), for example.
To calculate the enrichment factor of the different analytes on aerosol particles (EFaer) relative to seawater (SW), here distinguished between SML and ULW, the atmospheric concentration of the analyte relative to the sodium concentration on the PM1 sample was divided by the analyte concentration relative to the sodium concentration in the corresponding SW sample using Eq. (3).
The EFaer calculation was limited by the availability of the analyte concentration in both matrices, i.e., PM1 and SML samples were collected simultaneously.
2.2.5 Statistical analysis
To investigate possible relationships between chemical, (micro)biological and physical parameters of the seawater samples (ULW, SML), listed in Fig. S3, a Pearson correlation analysis was performed. Figures S4–S7 show the matrix of parameters when either ULW or SML samples of the dissolved or particulate fraction are considered. The correlation coefficient (R), the number of samples examined (n) and the p value were used to validate the significance of the correlation. In particular, the p value as a test for statistical hypothesis in research areas must be considered when defining statistical relevance (Bhattacharya and Habtzghi, 2002; Perezgonzalez, 2015). The statistical parameters for the performed analysis of the ULW and SML samples are defined as follows. For the ULW samples, the statistical relevance of a relationship is defined if n≥4 (nmax=13), R≥0.6 and p value ≤0.05 (Perezgonzalez, 2015). We consider a “trend” to be valid if n≥4 (nmax=13), R≥0.4 and p value ≥0.05. Due to the limited number of SML samples, p values were always ≥0.05, so we could not define a statistical relevance but considered a “trend” to be valid if n≥4 (nmax=6) and R≥0.4.
3.1 Seawater and SML samples
3.1.1 Lipids and lipid classes in the particulate fraction
The particulate lipids showed a certain variance during the campaign, as shown in Fig. 1. For the ULW samples, the concentration of ∑PL was between 36.4 and 93.5 µg L−1, for the SML samples between 61.0 and 118.1 µg L−1. The measured total lipid concentrations are within the concentration range of the previous measurement studies in different oceanic regions (Frka et al., 2011; Gašparović et al., 2014; Stolle et al., 2019). Within the PL, the lipid classes FFA (ULW: 5.4–14.0 µg L−1; SML: 16.1–36.5 µg L−1) and PP (ULW: 15.2–54.9 µg L−1; SML: 17.6–37.4 µg L−1) had high concentrations in seawater, while other lipid classes such as TG (<5.8 µg L−1) and ST (<2.4 µg L−1) had concentrations lowered by a factor of 4–23. Another interesting feature is the opposite abundance of the two phospholipids PE and PG. On sampling days when the PE concentration was high (e.g., 3 October 2017: 41.8 µg L−1; 4 October 2017: 41.9 µg L−1), the PG concentration, however, was lower (3 October 2017: 8.8 µg L−1; 4 October 2017: 12.6 µg L−1; see Fig. 1), whereas towards the end of the campaign, the concentration of PE decreased by a factor of 4–5 (e.g., 7 October 2017: 7.6 µg L−1; 9 October 2017: 9.8 µg L−1), while the concentration of PG (7 October 2017: 14.8 µg L−1; 9 October 2017: 14.4 µg L−1) increased by a factor of up to 2. The PE PG ratio calculated from these data varied throughout the campaign with increasing values towards the middle of the campaign (maxima on 3 October 2017 and 4 October 2017) and decreasing values towards the end (Table S4), following the same trend as the total bacteria number (TCN; Table S3). This indicates a change in the lipid dominant biological contributions, with bacterial sources dominating in the first part and especially in the middle of the campaign. However, in the last part of the campaign (after 5 October 2017) contributions to the lipid pool were rather dominated by phytoplankton. These differences between bacterial and phytoplankton sources are not reflected in the total lipid concentrations, because degradation products such as FFA also contribute to total lipids with a high proportion (Fig. 1). For this reason, neither bacterial nor phytoplankton sources alone are the controlling drivers for the total lipid concentration, at least in the short term.
3.1.2 Lipids and lipid classes in the dissolved fraction
Compared to the particulate fraction, higher concentrations of total dissolved lipids were detected by a factor between 1.1 and 1.4 with ∑DL: 39.8–128.5 µg L−1 in the ULW and with ∑DL: 55.7–121.5 µg L−1 in the SML samples (Fig. 2). The maximum concentrations here were also a factor of 1.3–1.4 higher than the total dissolved lipid concentrations reported by Frka et al. (2011) in the Mediterranean semi-enclosed temperate Adriatic sea (∑DL: 7.5–92.2 µg L−1).
In contrast to the particulate lipids, HC showed the highest concentration and variation within the lipids as it varied between 6.6 up to 64.0 µg L−1 in the ULW and in the SML between 9.2–49.6 µg L−1 (Fig. 2). HC can have both anthropogenic and natural sources (Scholz-Böttcher et al., 2009). Shorter n-alkanes (such as 2-nonadecane) may have additional sources as mature organic matter or petroleum contamination, but the main source of the investigated HC surrogate (2-nonadecane) is marine phytoplankton (Scholz-Böttcher et al., 2009, and references therein).
Phospholipids, especially PE and PG, and FFA, which dominated the particulate lipids, showed lower concentrations by a factor of 1.1–2.1 within the total dissolved lipids. In contrast to the particulate fraction, both phospholipids (PE and PG) showed similar concentrations and percentages in the dissolved fraction (Fig. 2).
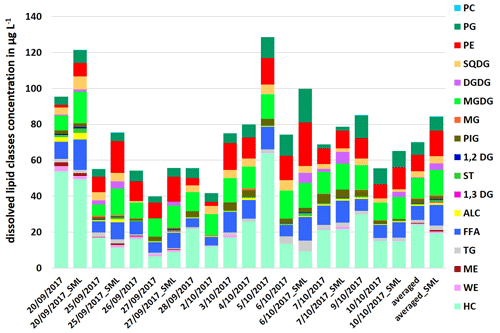
Figure 2Concentration of individual dissolved lipid classes in the ULW (sampling date) and the SML (sampling date_SML) samples along the campaign and as an averaged value in µg L−1.
The percentage composition of the total dissolved lipids is in good agreement with the literature (Goutx et al., 2009; Marić et al., 2013). Altogether, our detailed analysis of the lipid classes shows that, although the concentrations of PL and DL are overall very similar, the composition of the lipids compared to the PL and DL groups is partly different. This indicates that different production and degradation mechanisms for DL and PL contribute to the respective lipid composition.
3.1.3 Lipolysis index
To evaluate the lipid degradative state and (bio)degradation processing, the LI (Eq. 1; Table S5) was calculated for the lipids in the seawater samples. The LI in the particulate fraction varied between 0.13–0.31 in the ULW and between 0.37–0.66 in the SML samples. In their study in the northwestern Mediterranean Sea, van Wambeke et al. (2001) reported LI values of particulate lipids (0.21–0.39) during the end of a phytoplankton bloom up to pre-oligotrophic conditions. In general, the LI indicated that the intact lipid classes were dominant compared to the degradation indices/metabolites for the particulate lipids and that the lipids were thus degraded only to a small extent. This coincides well with the low concentrations of chlorophyll degradation products (PIG), suggesting that only moderate grazing took place and the (pigment-containing) organisms were fresh and in healthy condition (van Pinxteren et al., 2020). However, on specific days, the LISML of PL was ≥0.5 (Table S5), indicating an increased OM lipid degradation and metabolite release in the SML compared to the ULW. A higher LI in the SML was also observed by Gašparović et al. (2014) in the East Atlantic Ocean and can be attributed to both bacterial and photochemical abiotic degradation (Christodoulou et al., 2009). The LI of DL (Table S5) varied between 0.13–0.53 in the ULW and between 0.20–0.48 in the SML samples, suggesting that the dissolved lipid classes were more resistant to degradation than the particulate lipids.
3.1.4 Pigments, nutrients and microbiological investigations in seawater
To further elucidate the biological production and degradation state of lipids, the lipid concentrations were related to a set of biological parameters, including indicators for autotrophic organisms (namely marine pigments, chl a, Nanoeucaryotes and Synechococcus-like cells) and TCN as a proxy for bacterial abundance. Altogether, the concentrations of pigments as well as autotrophic and heterotrophic cells indicated an oligotrophic system (detailed values in Tables S2 and S3; further information is given in van Pinxteren et al., 2020). In addition, the chl a concentration in seawater increased from 0.11 to 0.60 µg L−1 (Table S2) during the campaign but was generally low compared to other subtropical/tropical regions or worldwide (Duhamel et al., 2019).
The pigment measurements of the bulk water indicated temporal changes in the composition of the community and an increasing trend in pigment concentration towards the end of the campaign. A correlation of total lipids with the abundances of chl a, Nanoeucaryotes and Synechococcus-like cells was not observed. However, with regard to specific pigments beyond chl a, a statistically relevant correlation between particulate PE and the pigment zeaxanthin with R=0.69 (p value =0.03, n=10) was found in the ULW (Fig. S8a). Zeaxanthin has been reported as a proxy for chlorophytes and cyanobacteria (Grant and Louda, 2010) and for some microalgae (Galasso et al., 2017, and references therein). Furthermore, the pigment fucoxanthin, a marker for diatoms (Descy et al., 2009), showed a trend with particulate FFA (R=0.53, p value =0.12, n=10) in ULW samples (Fig. S8b). The observed correlations and trends of zeaxanthin and fucoxanthin with individual particulate lipid classes pointed to a contribution of chlorophytes, cyanobacteria and diatoms within the lipid pool in our study.
We observed a positive and statistically relevant correlation between PE and TCN in the dissolved fraction of ULW samples (R=0.79, p value =0.006, n=10, Fig. S9a). We also found a positive correlation between the particulate PE and TCN in the ULW (R=0.72, p value =0.02, n=10); a similar trend was noticed for PE and TCN in the SML (R=0.64, p value =0.36, n=4) (Fig. S9b). The contribution of bacteria to the PE pool most likely results from the fact that PE is part of the bacterial membrane (Stillwell, 2016; Gašparović et al., 2018). Additional indications for bacteria influencing the lipid pool result from a negative correlation between the lipolysis index of total particulate lipids (LIPL) and TCN in the ULW and the SML samples. In the ULW, a correlation between LIPL and TCN with (p value =0.02, n=10) (Fig. S9c) was observed. In the SML (Fig. S9c), a similar trend was noticeable for LIPL and TCN (, p value =0.13, n=4). These relationships may result from the passive contribution (higher cell abundance and thus higher concentration of PE) of TCN to the phospholipids pool via PE. Phospholipids contribute to LI as part of the intact lipids (Sect. 2.2.3, Eq. 1), which leads to a negative correlation with LIPL. On the other hand, the metabolites could be actively taken up by bacteria, which most likely happens when more bacteria are present. Due to the low abundance of Synechococcus-like cells, we assume that most bacteria counted as TCN are heterotrophic and have taken up the metabolites. However, it remains unclear whether the bacteria have a passive (i.e., via membrane) or active (i.e., metabolism of the lipid “metabolites”) effect on the observed correlation between LIPL and TCN. It is most likely that bacteria have influenced the lipid pool which is consistent with the results obtained from the lipid composition.
3.2 Transfer of lipids from the oceans
3.2.1 The comparability of the different marine matrices (seawater and aerosol particles)
The concerted measurements performed here also included spot samplings in the ocean (ULW, SML) during the sampling period of PM1 aerosol particles at the CVAO (24 h). The air masses arriving at the CVAO often followed the water current (Peña-Izquierdo et al., 2012; van Pinxteren et al., 2017) and suggest an enhanced link between the upper ocean and the aerosol particles, as mainly winds drive the ocean currents in the upper 100 m of the ocean. The backward trajectories, as well as the concentrations of inorganic ions and mineral dust tracers on the aerosol particles measured during the campaign, suggested a predominant marine origin with low to medium dust influences (Triesch et al., 2021; van Pinxteren et al., 2020).
3.2.2 Enrichment in the SML
The EFSML was calculated using Eq. (2) to compare the concentration of the lipid classes in the SML samples with the ones in the ULW samples. The EFSML of the total lipids and of lipid class representatives in the particulate and dissolved fraction is listed in Table S6. For the total lipids in the particulate fraction, the EFSML varied between 1.0–1.7 (averaged EFSML(∑PL): 1.4), whereas in the dissolved fraction it varied between 1.1–1.4 (averaged EFSML(∑DL): 1.3). The EFSML of the total lipids are therefore quite similar for PL and DL.
A major aspect contributing to lipid enrichment in the SML might be explained by the physicochemical properties of the respective lipid classes such as the surface activity (Table S7). The parameters describing this characteristic, i.e., the density, the partitioning coefficient between octanol and water (KOW), and the topological polar surface area (TPSA), were compared with lipid enrichment. As shown in Table S7, the nonpolar lipids such as FFA and ALC have a higher surface enrichment potential compared to the more polar glycolipids and phospholipids, but a correlation between the enrichment of the lipids and the surface activity was absent. In the dissolved fraction neither such a gradation of enrichments at the surface nor a correlation between the enrichment and parameters describing the surface activity were visible. As Table S6 shows, similar EFSML values were found in the dissolved fraction for the individual lipid classes (EFSML: 1.5 (FFA), 1.7 (ALC) and 1.6 (PP)). A comparison of lipid enrichment with other OM compounds showed that the SML enrichment of lipids seemed to be less pronounced in contrast to other organic species such as amino acids (Reinthaler et al., 2008; Triesch et al., 2021), despite the high surface activity of the lipids (Burrows et al., 2014, and references therein). It has to be considered that the SML described here represents a layer with a thickness of about 100 µm (van Pinxteren et al., 2017), and therefore gradients within this layer (e.g., an enhanced enrichment of surfactants only in the top layer of a few µm) cannot be considered here. The fact that other (less surface active) compounds are more enriched in the SML (upper 100 µm) underlines the need to consider additional parameters to describe the SML enrichment of lipids in the ambient marine environment.
To this end, besides the physical processes leading to SML enrichment, the potential importance of OM producers and degraders (bacteria, phytoplankton and their released metabolites) were further investigated. Regarding the enrichment in the SML within the lipid classes or both fractions (the dissolved and particulate one), clearer differences were found when looking at the individual lipid classes. The bacterial marker PE was enriched in the SML in the DL and PL fractions with an EFSML(PE) of 1.6 (PF) and of 2.1 (DF). In contrast, the phytoplankton marker PG was always depleted in the SML (EFSML<1) in the PL and mostly enriched in the DL (averaged EFSML(PG) of 1.3). This is consistent with the observed permanent abundance and the slight enrichment of TCN and may indicate an enhanced bacterial activity in the SML. Furthermore, the degradation lipid class FFA showed high enrichments in the SML (averaged EFSML(FFA): of 3.1 (PL) and 1.5 (DL)). These high concentrations and enrichments point to an enhanced biodegradation in the SML, which is consistent with previous observations (Frka et al., 2011; Gašparović et al., 2018) that lipids are more degraded in the SML (high LI) than in the ULW. Besides the increased abundance of microbial cells, this may also be due to a different microbial community composition between the SML and the ULW and thus to different functional diversity (Cunliffe et al., 2011). The metabolic reserve lipids, represented by TG, showed the highest variability of enrichment in the SML along the campaign in the particulate fraction. In this fraction, EFSML(TG) varied between 0.3 and 4.4, resulting in an averaged enrichment of 2.3. The enrichment in the lipid classes of the dissolved fraction was less pronounced and always showed an opposite trend to PL; i.e., if the TG was highly enriched in the PL, it would have been less enriched in the DL and vice versa. This indicates that lipid reserves such as TG are stored within the particulate pool and upon dissolution become part of the dissolved pool.
Altogether, our results indicate that physicochemical descriptors alone, which are related to the surface activity of the lipids, are not sufficient to describe the SML enrichment of the lipids, at least not in the top 100 µm. In situ formation and degradation by phytoplankton and mainly bacterial processes, as shown here from the lipid classes patterns, also contribute to the abundance and SML enrichment of lipids in the ambient marine seawater.
3.2.3 Measured PM1 aerosol particle composition
Up to now, the discussion about lipids on (marine) aerosol particles has only covered distinct classes of lipids (mostly fatty acids). Given this fact, this work firstly presents a comprehensive analysis of several lipid classes on marine aerosol particles. The atmospheric concentration of the total lipids in the PM1 samples at the CVAO varied between 75.2 and 219.5 ng m−3 (average 119.9 ng m−3), as shown in Fig. 3. It was 18.5 ng m−3 (8.7–33.9 ng m−3) for FFA and 6.3 ng m−3 (3.4–9.8 ng m−3) for ALC at the CVAO. These values are in good agreement with Kawamura et al. (2003), who reported atmospheric concentrations between 0.19–23 ng m−3 (average 2.0 ng m−3) for ALC and between 2.5–38 ng m−3 (average 14 ng m−3) for FAA on marine aerosol particles from the western North Pacific. Other than that, Mochida et al. (2002) observed atmospheric concentration between 0.8–24 ng m−3 for saturated fatty acids (C14–C19) on marine aerosol particles collected over the northern Pacific. A high percentage contribution of the lipid classes TG, FFA and ALC (26.3 %–64.0 %, average 39.8 %) to the total lipids was observed. In particular, high percentages for TG (11.9 %–29.1 %, average 18.8 %) and FFA (8.4 %–31.2 %, average 15.4 %) were noticeable.
Compared to the seawater lipids, the atmospheric composition showed the same classes of lipids with an increased consistency of the DL composition (high contribution of HC and lower contributions of PP).
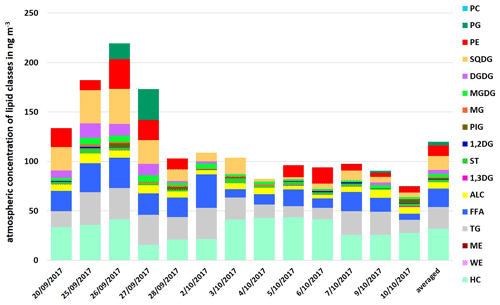
Figure 3Atmospheric concentration of individual lipid classes in PM1 aerosol particle samples and as an average at the CVAO in ng m−3.
However, although the atmospheric concentration of phospholipids was lower, PE was found to be more concentrated than PG, with only one exception on 27 September 2017 (Fig. 3). Since heterotrophic bacteria are reported as a dominant source of PE (Michaud et al., 2018), this suggests that (i) bacteria, possibly transported from the ocean into the atmosphere, contribute PE on the aerosol particles and/ or (ii) PE is directly transferred from the ocean into the atmosphere, likely via bubble bursting.
3.2.4 Transfer of lipid classes from the ocean to the aerosol particles
A frequently applied parameter to quantify the transfer of OM from the ocean into the atmosphere is the EFaer (e.g., Russell et al., 2010; van Pinxteren et al., 2017; Triesch et al., 2021). According to Eq. (3), the EFaer(TL) was calculated based on the dissolved total lipids in the SML and varied between 9×104 and 7×105 with an average value of 3×105 (Fig. 4, Table S8). The EFaer based on the particulate total lipids in the SML was with an average of 2×105 very similar to the EFaer of the dissolved total lipids (3×105) as discussed in the Supplement (Table S8). The data reported in the literature for enrichment factors of organic carbon or groups of OM on aerosol particles often originate from laboratory experiments, e.g., using controlled artificial bubbling units (Quinn et al., 2015, and references therein). Rastelli et al. (2017) determined the enrichment of lipids as a sum parameter on submicrometer aerosol particles compared to seawater in a bubble-bursting experimental set-up and found an EFaer of 1×105. The good agreement of the EFaer(TL) derived from the ambient measurements reported here with the EFaer derived from laboratory experiments under controlled conditions by Rastelli et al. (2017) indicates that the transfer of lipids from the SML to the aerosol phase under ambient conditions is consistent with processes described in laboratory studies.
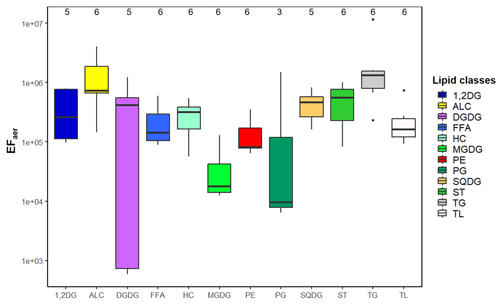
Figure 4Boxplot of the enrichment factor aerosol (EFaer) of the individual lipid classes and total lipids (TL) at the CVAO including the median and the 25th and 75th percentiles; a further explanation is given in Fig. S10.
The mean EFaer(TL) calculated and based on the ULW concentration is 3.4×105 and thus very similar to the mean EFaer(TL) based on the SML concentrations (2.6×105). This can be attributed to the fact that the lipid concentrations in the ULW and SML were in the same concentration range, resulting in a comparatively low enrichment in the SML (EFSML: 1.0–1.7; Sect. 3.2.2).
All in all, the EFaer of the total lipids was about 1 to 2 orders of magnitude higher than the EFaer of free amino acids (4×102–3×104) on submicrometer aerosol particles measured during the same campaign (Triesch et al., 2021) and underlining the preferred transfer of lipids from the ocean into the atmosphere. In contrast to the SML enrichment, the higher enrichment of the lipids on the aerosol particles observed here corresponds to the high surface activity of the lipids and the preferred adsorption to (bubble) surfaces resulting in a strong sea-to-air-transfer (Tervahattu et al., 2002; Facchini et al., 2008; Cochran et al., 2016a; Schmitt-Kopplin et al., 2012; Rastelli et al., 2017), and their possible association with other compounds promoting co-aerosolization processes (Quinn et al., 2015; Hoffman and Duce, 1976; Rastelli et al., 2017). Further possible transport mechanisms are discussed in Sect. 3.2.4.
A gradient regarding the EFaer of the individual lipid classes was found, showing that some of them were enriched to a larger extent than others (Fig. 4, Table S9). Such differences between the lipid classes have not been reported so far because the lipids were mainly measured as a sum (Rastelli et al., 2017), or only a specific lipid class (such as FFA, HC) was investigated (Cochran et al., 2016b; Marty et al., 1979). In our data set we observed that TG (EFaer(TG): 3×106) followed by ALC were the most enriched (EFaer(ALC): 1×106), while MGDG showed a lower enrichment with the EFaer(MGDG): 4×104. For the SML enrichment, the parameters describing the surface activity (density, KOW and TPSA; Table S7) were compared with lipid enrichment. Lipid classes with a comparatively low surfactant activity (in relation to the TPSA value of the lipid class; Table S7) including PP and GL showed relatively lower enrichments (EFaer(PP): 2×105 and EFaer(GL): 3×105) compared to highly enriched ALC (EFaer(ALC): 1×106). Furthermore, a statistically relevant correlation (R2=0.45, p=0.028) was found between the log KOW and the EFaer of the individual lipid classes (Fig. S11), indicating that the compounds with higher log KOW and thus a higher lipophilicity are preferably enriched on the aerosol particles. For example, TG with the highest log KOW value of 25.5 (Table S7) was observed to have the highest enrichment on aerosol particles (EFaer(TG): 3×106). In contrast, lipid classes with lower log KOW values such as MGDG (log KOW(MGDG): −3.5) were characterized by lower enrichments (EFaer(MGDG): 4×104). The compounds that are highly enriched in the aerosol phase only partially correspond to their respective enrichment in the SML. TG and ALC showed a high enrichment in both the SML and on the aerosol particles. However, FFA that showed a pronounced SML enrichment in PL (EFSML(FFA): 3.1) and exhibited only a medium enrichment on the aerosol particles compared to other lipid groups.
It needs to be emphasized that the calculated EFaer provides the quantitative description of the transfer from the ocean into the atmosphere but does not consider additional formation or degradation pathways of the lipids on the aerosol particles, including biological or photochemical atmospheric reactions and a transport from other than marine sources. In agreement with the results of the SML enrichment, these results suggest that additional processes such as biotic formation and degradation influence the lipid abundance on the aerosol particles. It has been reported that microorganisms (Rastelli et al., 2017) and especially bacteria (Michaud et al., 2018) can be transported from the ocean into the atmosphere. Bacteria can be transferred to marine aerosol particles. Here, besides passive contribution (i.e., providing lipids to aerosol particles upon cell disintegration), bacteria may also actively influence the OM composition of aerosols (i.e., lipid production or degradation). However, the extent of this passive and especially of potentially active bacteria contribution to the lipid pool of aerosols warrants further studies. In addition, photochemical oxidation processes can take place in the atmosphere, i.e., the conversion of FFA to ALC (Bikkina et al., 2019).
Overall, the detailed measurements of the lipid classes (within the concerted measurements) together with additional parameters showed that although lipids are the largest OM species on aerosols, which is in line with their high surface activity, additional biological processes influence the lipid composition. This needs to be further studied and considered in OM transfer models. More recent models recognized that the OM transfer must be modeled by including the individual OM groups (like lipids) rather than phytoplankton tracers like chl a (Burrows et al., 2014). Indeed, these models describe the OM transfer on the basis of their physicochemical properties, but our data suggest that in the ambient marine environment, additional in situ formation and degradation must also be considered in order to fully address the OM abundance in general and the lipid abundance in particular.
3.2.5 Discussion of possible transfer mechanisms
The transfer of the dissolved and particulate OM from the ocean into the atmosphere probably occurs via bubble bursting, whereby bubbles rising through the water column absorb OM and, when bursting at the surface, release the OM to the aerosol particles via jet and film drops (Quinn et al., 2015), and references therein). The finding that both classes of lipids (DL and PL) are found on the aerosol particles (Figs. 5, S12) indicates that both types of lipids can be transferred from the seawater to the aerosol particles, e.g., via bubble bursting. A differentiation of the contribution of the dissolved and particulate lipid fractions in seawater to the formation of the aerosol particles was therefore not possible here.
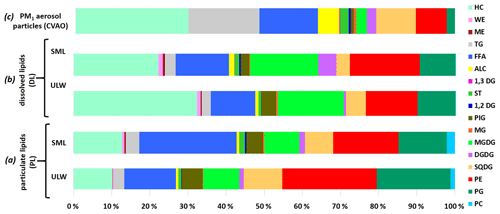
Figure 5The percentage contribution of the individual lipid classes to the total lipids in the (a) particulate and (b) dissolved fraction in seawater (differentiation between ULW and SML) and on (c) PM1 aerosol particles at the CVAO.
However, regarding the process of the OM absorption on the bubbles in more detail, the OM absorbed on the bubble can be distributed either towards the gas or aqueous phase or can preferably reside within the bubble interface. To conceptually address the distribution of the OM towards the bubble–water interface, we calculated the adsorption coefficient related to air (Ka) after Kelly et al. (2004) and additionally an adsorption coefficient of the analytes related to water (Kaq). The calculation included the measured SML concentrations, Henry's law constants and the gas-phase saturation vapor pressure as explained and discussed in detail in Table S10. The comparison of the calculated Ka and Kaq values can give a hint on the distribution of lipids at the bubble–air–water interface. When Kaq>>Ka (Fig. S17a), the analyte should be preferably distributed (from water) into air (inside the bubble). When Ka>>Kaq (Fig. S17b) in turn, the analyte should be preferably distributed (from air) into water while the analyte should be preferably distributed within the bubble interface when Kaq∼Ka (Fig. S17c). Our data set showed that the analytes that had similar Ka and Kaq values (Table S10), namely TG and ALC, had the highest EFaer (EFaer(TG): 3×106 and EFaer(ALC): 1×106). This indicates that analytes, which are preferably distributed within the interface of the bubble, are transferred to the aerosol particles to a larger extent via the bubble bursting process, probably due to the higher stickiness to the interface (Fig. S18). For MGDG, the lipid class with the lowest EFaer (4×104), the observed ratio was Ka>>Kaq, meaning that MGDG was preferably distributed into water. For the other lipid classes, however, we did not find such a connection between the adsorption coefficients (Kaq, Ka) and the aerosol enrichment (EFaer). Nevertheless, the hypothesis that the transfer and enrichment of the lipids are related to the distribution of compounds within the bubble–air–water interface, as observed for the lipid classes with extreme EFaer, should be further investigated, preferably in controlled laboratory experiments.
3.3 Connection between lipids and INP activity in seawater
One main feature of biological components in general is their potential ability to contribute to ice nucleation and act as INPs in the atmosphere (Šantl-Temkiv et al., 2019, and references therein). To identify potential connections between lipid classes and INP activity in seawater, a statistical analysis was performed as described in Sect. 2.2.5. As shown in detail in Gong et al. (2020), all samples collected for the present study contained both SML and ULW INPs with concentrations of ∼200 L−1 at temperatures of about −10 ∘C (Fig. S15), increasing to 107 L−1 at ∘C. The existence of INPs that are already ice-active at temperatures above −15 ∘C indicated the presence of biogenic INPs (Kanji et al., 2017; Šantl-Temkiv et al., 2019).
Both lipid fractions of the SML samples showed a positive trend towards the INP concentrations measured at −10 ∘C (R=0.72, p value =0.28, n=4 for the total PL and R=0.69, p value =0.31, n=4 for the total DL; Fig. S13a and b). This connection suggests the involvement of lipids in biogenic INPs. Furthermore, the lipid classes which had shown a relationship with autotrophic or heterotrophic organisms, namely PE and FFA (Sect. 3.1.4), were investigated for their INP relationship. The relationship between PE and INPs with regard to ULW cannot be discussed here, as the criteria defined in Sect. 2.2.5 were not met (e.g., not enough matching data points were available). But a trend was found between the SML particulate PE and INP measurements at −10 ∘C (R=0.95, p value =0.06, n=4, Fig. S13c). Similar relations between lipids and the INP activity have been reported previously (Govindarajan and Lindow, 1988; Palaiomylitou et al., 1998; DeMott et al., 2018). Palaiomylitou et al. (1998) reported that ice nucleation proteins were associated with phospholipids and showed that phospholipids, especially PE, not only contribute to the increased overall activity but also to the production of ice nuclei active at higher temperatures. To further test the biogenic INP activity, we analyzed the INP activity of the seawater samples before and after heating (95 ∘C for 1 h), since biogenic, especially proteinogenic, compounds are deactivated when heated to 100 ∘C (Šantl-Temkiv et al., 2019). It could be shown that a large proportion of INPs that were active between −10 and −15 ∘C lost their ice activity after the heating procedure (Fig. S15). As mentioned above, the deactivation of the INP function by heating is often associated with proteins. However, it has been shown that ice nucleating proteins have a connection to lipids and that interactions with membrane lipids, especially PE, are needed to maintain the conformational structure and functional activity of many membrane-bound proteins (Govindarajan and Lindow, 1988; Palaiomylitou et al., 1998). For this reason, the lipids might have a driving function in the INP activity of biogenic INPs. In the SML samples, trends between INP measurements at −10 ∘C and the particulate FFA (R=0.84, p value =0.16, n=4, Fig. S14a) and dissolved FFA (R=0.63, p value =0.37, n=4, Fig. S14b) were observed. Moreover, a trend was found between the particulate FFA in the ULW and the INP measurements at −15 ∘C (R=0.64, p value =0.025, n=12, Fig. S14c). DeMott et al. (2018) reported that the ice nucleation by particles containing long-chain fatty acids in a crystalline phase was relevant for the freezing of sea spray aerosols. Burrows et al. (2013) suggested that marine biogenic INPs most probably played a dominant role in the INP concentrations studied in near-surface air over the Southern Ocean. Wilson et al. (2015) proposed that there are ice-active macromolecules in the OM of the SML. Moreover, they pointed out that global model simulations of marine organic aerosol in connection with their measurements indicated that marine OM might be an important source of INPs in remote marine environments, e.g., the Southern Ocean, North Pacific Ocean and North Atlantic Ocean (Wilson et al., 2015, and references therein). The relationships presented here between the lipids in general and in particular the lipid classes with assigned biological context (PE, FFA) and INP activity at higher temperatures (−10, −15 ∘C) in the ambient SML indicate that lipids in the tropical North Atlantic Ocean have the potential to contribute to (biogenic) INP activity when transferred into the atmosphere. However, it remains unclear to what extent INPs transferred from the ocean into the atmosphere contribute to the INP pool in the atmosphere, since further studies have identified other sources of INPs besides sea spray aerosol (Gong et al., 2020, and references therein).
Concerted measurements of lipids were performed in proximity to the Cabo Verde islands to compare the concentration of specific lipid classes on submicrometer aerosol particles and in the dissolved and particulate fraction of seawater (ULW and SML). The analysis of lipid classes in seawater showed that, although concentrations in the particulate and dissolved fraction are generally very similar, the contribution of lipids within both fractions differed. This suggests that different production and degradation mechanisms for DL and PL contribute to the respective lipid composition. On the aerosol particles, the lipid composition resembles the lipid composition of the dissolved fraction in seawater. The phytoplankton groups chlorophytes, cyanobacteria and diatoms probably influence the lipid abundance as shown by pigment measurements. Our results indicate that, besides phytoplankton, bacteria also play an important role in lipid abundance in the oligotrophic North Atlantic, as shown by the PE PG ratio and the abundance (and slight SML enrichment) of TCN. The concentration and enrichment of lipids in the ambient SML are not related to their physicochemical properties describing the surface activity, at least not in the short term, probably due to parallel in situ formation and degradation processes. This is underlined by the fact that lipids in the top 100 µm of the SML are not as highly enriched as other (less surface active) compounds, such as amino acids.
The EFaer agrees with the considerations from modeling and laboratory studies that, among the marine OM groups, lipids are the most highly enriched compounds and indicates that the transfer of lipids from the SML to the aerosol phase in the complex marine field is consistent with processes described in laboratory studies. In addition, the EFaer of lipids was one to two orders of magnitude higher than the EFaer of the less surface-active amino acids previously reported. In terms of the individual lipid groups on the aerosol particles, a statistically significant correlation (R2=0.45, p=0.028) between EFaer and lipophilicity (expressed by the KOW value) was observed, which was not present for the SML. In general, however, the parameters representing the surface activity of the lipid classes (density, KOW value and TPSA) were not sufficient to describe their transfer to the aerosol particles. The fact that bacteria are clearly involved in lipid abundance underlines that models using chl a are not enough to describe OM in general. In addition, physicochemical OM properties such as surface activity, are not sufficient to describe lipid abundance in the complex marine environment. Further processes such as biotic formation and degradation, as shown by the investigation of the individual lipid classes, contribute to lipid abundance in seawater, the SML and on aerosol particles in the marine environment and must be included in the consideration of lipid transfer and finally in OM transfer models. Beyond that, our data suggest that the enrichment of the lipid classes on aerosol particles may be related to the distribution of the lipids on their respective adsorption coefficients in water (Kaq) and in air (Ka). Compounds which are preferably arranged within the bubble interface (Kaq∼Ka), namely TG and ALC, are transferred to the aerosol particles to the highest extend. Finally, our results showed that lipids had the potential to contribute to (biogenic) INP activity when transferred into the atmosphere.
Altogether, we showed that the diverse group of lipids represent an important and complex OM group in seawater and on marine aerosol particles. To the best of the author's knowledge, the present study is the first to analyze several lipid classes simultaneously in seawater including the ULW and the SML and on submicrometer aerosol particles (PM1) in such detail to obtain indications on their sources and sea-air linkage in the marine environment.
The open-source statistic software R (R-Core Team, 2018) was used for the Pearson correlation analysis (Friendly, 2002). Data processing and visualization were done with the packages of the “tidyverse” (Wickham, 2017) and with Microsoft Office Standard 2019 (Excel).
The data are available through the World Data Centre PANGAEA under the following link: https://doi.org/10.1594/PANGAEA.921832 (Triesch et al., 2020).
The supplement related to this article is available online at: https://doi.org/10.5194/acp-21-4267-2021-supplement.
NT wrote the paper with contributions from MvP, SF, BG, CS, TS, EHH, XG, HW, DSB and HH. NT and MvP performed the field sampling as part of the MarParCloud campaign team. NT supported by TS did the sample preparation for the lipid measurements and NT, together with SF and BG, performed the lipid measurements. The lipid data evaluation was done by NT, SF and BG in consultation with MvP and HH. CS and DSB performed the (micro)biological and nutrient investigations in seawater and XG and HW the measurements of INP activity. The statistical analysis was done by NT with support of TS, CS, MvP and HH. The introduction and implementation of the adsorption coefficients were carried out by NT with the support of EHH and in consultation with MvP and HH. All authors discussed the results and further analysis after the campaign. All co-authors proofread and commented on the paper.
The authors declare that they have no conflict of interest.
This article is part of the special issue “Marine organic matter: from biological production in the ocean to organic aerosol particles and marine clouds (ACP/OS inter-journal SI)”. It is not associated with a conference.
Sanja Frka and Blaženka Gašparović acknowledge the Croatian Science Foundation for the full support under the Croatian Science Foundation project IP-2018-01-3105 (BiREADI). The authors also thank Susanne Fuchs, Anett Dietze, René Rabe and Anke Rödger for providing additional data and filter samples and all MarParCloud and MARSU project partners for a good cooperation and support. The authors thank Kerstin Lerche from the Helmholtz-Zentrum für Umweltforschung GmbH UFZ in Magdeburg for the pigment measurements. Additional thanks go to Joanna Waniek, Jenny Jeschek and Christian Burmeister for analyzing inorganic nutrients and Katja Kaeding for flow cytometry measurements. We further acknowledge the professional support provided by the Ocean Science Centre Mindelo (OSCM) and the Instituto do Mar (IMar).
This work was funded by the Leibniz Association SAW for the project “marine biological production, organic aerosol particles and marine clouds: a process chain (MarPar-Cloud)” (grant no. SAW-2016-TROPOS-2) and within the Research and Innovationn Staff Exchange EU project MARSU (grant no. 69089).
The publication of this article was funded by the Open Access Fund of the Leibniz Association.
This paper was edited by Kimitaka Kawamura and reviewed by three anonymous referees.
Arts, M. T., Ackman, R. G., and Holub, B. J.: “Essential fatty acids” in aquatic ecosystems: a crucial link between diet and human health and evolution, Can. J. Fish. Aquat. Sci., 58, 122–137, https://doi.org/10.1139/f00-224, 2001.
Barati, F., Yao, Q., and Asa-Awuku, A. A.: Insight into the Role of Water-Soluble Organic Solvents for the Cloud Condensation Nuclei Activation of Cholesterol, ACS Earth Space Chem., 3, 1697–1705, https://doi.org/10.1021/acsearthspacechem.9b00161, 2019.
Becker, K. W., Collins, J. R., Durham, B. P., Groussman, R. D., White, A. E., Fredricks, H. F., Ossolinski, J. E., Repeta, D. J., Carini, P., Armbrust, E. V., and Van Mooy, B. A. S.: Daily changes in phytoplankton lipidomes reveal mechanisms of energy storage in the open ocean, Nat. Commun., 9, 5179, https://doi.org/10.1038/s41467-018-07346-z, 2018.
Bhattacharya, B. and Habtzghi, D.: Median of the p Value under the Alternative Hypothesis, Am. Stat., 56, 202–206, 2002.
Bikkina, P., Kawamura, K., Bikkina, S., Kunwar, B., Tanaka, K., and Suzuki, K.: Hydroxy Fatty Acids in Remote Marine Aerosols over the Pacific Ocean: Impact of Biological Activity and Wind Speed, ACS Earth Space Chem., 3, 366–379, https://doi.org/10.1021/acsearthspacechem.8b00161, 2019.
Bligh, E. G. and Dyer, W. J.: A rapid method of total lipid extraction and purification, Can. J. Biochem. Phys., 37, 911–917, 1959.
Burrows, S. M., Hoose, C., Pöschl, U., and Lawrence, M. G.: Ice nuclei in marine air: biogenic particles or dust?, Atmos. Chem. Phys., 13, 245–267, https://doi.org/10.5194/acp-13-245-2013, 2013.
Burrows, S. M., Ogunro, O., Frossard, A. A., Russell, L. M., Rasch, P. J., and Elliott, S. M.: A physically based framework for modeling the organic fractionation of sea spray aerosol from bubble film Langmuir equilibria, Atmos. Chem. Phys., 14, 13601–13629, https://doi.org/10.5194/acp-14-13601-2014, 2014.
Carpenter, L. J., Fleming, Z. L., Read, K. A., Lee, J. D., Moller, S. J., Hopkins, J. R., Purvis, R. M., Lewis, A. C., Muller, K., Heinold, B., Herrmann, H., Fomba, K. W., van Pinxteren, D., Muller, C., Tegen, I., Wiedensohler, A., Muller, T., Niedermeier, N., Achterberg, E. P., Patey, M. D., Kozlova, E. A., Heimann, M., Heard, D. E., Plane, J. M. C., Mahajan, A., Oetjen, H., Ingham, T., Stone, D., Whalley, L. K., Evans, M. J., Pilling, M. J., Leigh, R. J., Monks, P. S., Karunaharan, A., Vaughan, S., Arnold, S. R., Tschritter, J., Pohler, D., Friess, U., Holla, R., Mendes, L. M., Lopez, H., Faria, B., Manning, A. J., and Wallace, D. W. R.: Seasonal characteristics of tropical marine boundary layer air measured at the Cape Verde Atmospheric Observatory, J. Atmos. Chem., 67, 87–140, https://doi.org/10.1007/s10874-011-9206-1, 2010.
Christodoulou, S., Marty, J.-C., Miquel, J.-C., Volkman, J. K., and Rontani, J.-F.: Use of lipids and their degradation products as biomarkers for carbon cycling in the northwestern Mediterranean Sea, Mar. Chem., 113, 25–40, https://doi.org/10.1016/j.marchem.2008.11.003, 2009.
Cochran, R. E., Jayarathne, T., Stone, E. A., and Grassian, V. H.: Selectivity Across the Interface: A Test of Surface Activity in the Composition of Organic-Enriched Aerosols from Bubble Bursting, J. Phys. Chem. Lett., 7, 1692–1696, https://doi.org/10.1021/acs.jpclett.6b00489, 2016a.
Cochran, R. E., Laskina, O., Jayarathne, T., Laskin, A., Laskin, J., Lin, P., Sultana, C., Lee, C., Moore, K. A., Cappa, C. D., Bertram, T. H., Prather, K. A., Grassian, V. H., and Stone, E. A.: Analysis of Organic Anionic Surfactants in Fine and Coarse Fractions of Freshly Emitted Sea Spray Aerosol, Environ. Sci. Technol., 50, 2477–2486, https://doi.org/10.1021/acs.est.5b04053, 2016b.
Cunliffe, M., Upstill-Goddard, R. C., and Murrell, J. C.: Microbiology of aquatic surface microlayers, FEMS Microbiol. Rev., 35, 233–246, https://doi.org/10.1111/j.1574-6976.2010.00246.x, 2011.
Cunliffe, M., Engel, A., Frka, S., Gašparović, B., Guitart, C., Murrell, J. C., Salter, M., Stolle, C., Upstill-Goddard, R., and Wurl, O.: Sea surface microlayers: A unified physicochemical and biological perspective of the air-ocean interface, Prog. Oceanogr., 109, 104–116, https://doi.org/10.1016/j.pocean.2012.08.004, 2013.
Cunliffe, M. A. W. O.: Guide to best practices to study the ocean's surface, Marine Biological Association of the United Kingdom for SCOR, Plymouth, UK, 118 pp., 2014.
DeMott, P. J., Mason, R. H., McCluskey, C. S., Hill, T. C. J., Perkins, R. J., Desyaterik, Y., Bertram, A. K., Trueblood, J. V., Grassian, V. H., Qiu, Y., Molinero, V., Tobo, Y., Sultana, C. M., Lee, C., and Prather, K. A.: Ice nucleation by particles containing long-chain fatty acids of relevance to freezing by sea spray aerosols, Environ. Sci. Process. Imp., 20, 1559–1569, https://doi.org/10.1039/C8EM00386F, 2018.
Derieux, S., Fillaux, J., and Saliot, A.: Lipid class and fatty acid distributions in particulate and dissolved fractions in the north Adriatic sea, Org. Geochem., 29, 1609–1621, https://doi.org/10.1016/S0146-6380(98)00089-8, 1998.
Descy, J.-P., Sarmento, H., and Higgins, H. W.: Variability of phytoplankton pigment ratios across aquatic environments, Eur. J. Phycol., 44, 319–330, https://doi.org/10.1080/09670260802618942, 2009.
Duhamel, S., Kim, E., Sprung, B., and Anderson, O. R.: Small pigmented eukaryotes play a major role in carbon cycling in the P-depleted western subtropical North Atlantic, which may be supported by mixotrophy, Limnol. Oceanogr., 64, 2424–2440, https://doi.org/10.1002/lno.11193, 2019.
Engel, A., Bange, H. W., Cunliffe, M., Burrows, S. M., Friedrichs, G., Galgani, L., Herrmann, H., Hertkorn, N., Johnson, M., Liss, P. S., Quinn, P. K., Schartau, M., Soloviev, A., Stolle, C., Upstill-Goddard, R. C., van Pinxteren, M., and Zäncker, B.: The Ocean's Vital Skin: Toward an Integrated Understanding of the Sea Surface Microlayer, Front. Mar. Sci., 4, 165, https://doi.org/10.3389/fmars.2017.00165, 2017.
Facchini, M. C., Rinaldi, M., Decesari, S., Carbone, C., Finessi, E., Mircea, M., Fuzzi, S., Ceburnis, D., Flanagan, R., Nilsson, E. D., de Leeuw, G., Martino, M., Woeltjen, J., and O'Dowd, C. D.: Primary submicron marine aerosol dominated by insoluble organic colloids and aggregates, Geophys. Res. Lett., 35, L17814, https://doi.org/10.1029/2008GL034210, 2008.
Fomba, K. W., Müller, K., van Pinxteren, D., Poulain, L., van Pinxteren, M., and Herrmann, H.: Long-term chemical characterization of tropical and marine aerosols at the Cape Verde Atmospheric Observatory (CVAO) from 2007 to 2011, Atmos. Chem. Phys., 14, 8883–8904, https://doi.org/10.5194/acp-14-8883-2014, 2014.
Friendly, M.: Corrgrams: Exploratory displays for correlation matrices, The American Statistician, 56, 316–324, 2002.
Frka, S., Gašparović, B., Marić, D., Godrijan, J., Djakovac, T., Vojvodić, V., Dautović, J., and Kozarac, Z.: Phytoplankton driven distribution of dissolved and particulate lipids in a semi-enclosed temperate sea (Mediterranean): Spring to summer situation, Estuar. Coast. Shelf Sci., 93, 290–304, https://doi.org/10.1016/j.ecss.2011.04.017, 2011.
Frka, S., Pogorzelski, S., Kozarac, Z., and Ćosović, B.: Physicochemical Signatures of Natural Sea Films from Middle Adriatic Stations, J. Phys. Chem. A, 116, 6552–6559, https://doi.org/10.1021/jp212430a, 2012.
Gagosian, R. B., Zafiriou, O. C., Peltzer, E. T., and Alford, J. B.: Lipids in aerosols from the tropical North Pacific: Temporal variability, J. Geophys. Res.-Oceans, 87, 11133–11144, https://doi.org/10.1029/JC087iC13p11133, 1982.
Galasso, C., Corinaldesi, C., and Sansone, C.: Carotenoids from Marine Organisms: Biological Functions and Industrial Applications, Antioxidants, 6, 96, https://doi.org/10.3390/antiox6040096, 2017.
Gantt, B., Meskhidze, N., Facchini, M. C., Rinaldi, M., Ceburnis, D., and O'Dowd, C. D.: Wind speed dependent size-resolved parameterization for the organic mass fraction of sea spray aerosol, Atmos. Chem. Phys., 11, 8777–8790, https://doi.org/10.5194/acp-11-8777-2011, 2011.
Gašparović, B., Godrijan, J., Frka, S., Tomažić, I., Penezić, A., Marić, D., Djakovac, T., Ivančić, I., Paliaga, P., Lyons, D., Precali, R., and Tepić, N.: Adaptation of marine plankton to environmental stress by glycolipid accumulation, Mar. Environ. Res., 92, 120–132, https://doi.org/10.1016/j.marenvres.2013.09.009, 2013.
Gašparović, B., Frka, S., Koch, B. P., Zhu, Z. Y., Bracher, A., Lechtenfeld, O. J., Neogi, S. B., Lara, R. J., and Kattner, G.: Factors influencing particulate lipid production in the East Atlantic Ocean, Deep Sea Res., 89, 56–67, https://doi.org/10.1016/j.dsr.2014.04.005, 2014.
Gašparović, B., Kazazić, S. P., Cvitešić, A., Penezić, A., and Frka, S.: Improved separation and analysis of glycolipids by Iatroscan thin-layer chromatography-flame ionization detection, J. Chromatogr. A, 1409, 259–267, https://doi.org/10.1016/j.chroma.2015.07.047, 2015.
Gašparović, B., Kazazić, S. P., Cvitešić, A., Penezić, A., and Frka, S.: Corrigendum to “Improved separation and analysis of glycolipids by Iatroscan thin-layer chromatography-flame ionization detection”, J. Chromatogr. A, 1521, 168–169, https://doi.org/10.1016/j.chroma.2017.09.038, 2017.
Gašparović, B., Penezić, A., Lampitt, R. S., Sudasinghe, N., and Schaub, T.: Phospholipids as a component of the oceanic phosphorus cycle, Mar. Chem., 205, 70–80, https://doi.org/10.1016/j.marchem.2018.08.002, 2018.
Gong, X., Wex, H., van Pinxteren, M., Triesch, N., Fomba, K. W., Lubitz, J., Stolle, C., Robinson, T.-B., Müller, T., Herrmann, H., and Stratmann, F.: Characterization of aerosol particles at Cabo Verde close to sea level and at the cloud level – Part 2: Ice-nucleating particles in air, cloud and seawater, Atmos. Chem. Phys., 20, 1451–1468, https://doi.org/10.5194/acp-20-1451-2020, 2020.
Goutx, M., Acquaviva, M., and Gérin, C.: Iatroscan-measured phospholipids from marine microalgae, bacteria and suspended particles. Inform-International news on fats, oils and related materials, Am. Oil Chem. Soc. Publ., 4, 516–517, 1993.
Goutx, M., Guigue, C., and Striby, L.: Triacylglycerol biodegradation experiment in marine environmental conditions: definition of a new lipolysis index, Org. Geochem., 34, 1465–1473, https://doi.org/10.1016/S0146-6380(03)00119-0, 2003.
Goutx, M., Guigue, C., D. Aritio D., Ghiglione, J. F., Pujo-Pay, M., Raybaud, V., Duflos, M., and Prieur, L.: Short term summer to autumn variability of dissolved lipid classes in the Ligurian sea (NW Mediterranean), Biogeosciences, 6, 1229–1246, https://doi.org/10.5194/bg-6-1229-2009, 2009.
Govindarajan, A. G. and Lindow, S. E.: Phospholipid requirement for expression of ice nuclei in Pseudomonas syringae and in vitro, J. Biol. Chem., 263, 9333–9338, 1988.
Grant, C. S. and Louda, J. W.: Microalgal pigment ratios in relation to light intensity: Implications for chemotaxonomy, Aquat. Biol., 11, 127–138, https://doi.org/10.3354/ab00298, 2010.
Grasshoff, K., Kremling, K., and Ehrhardt, M.: Methods of Seawater Analysis, 3rd edition, Wiley-VCH, Weinheim, Germany, 1999.
Guschina, I. A. and Harwood, J. L.: Algal lipids and effect of the environment on their biochemistry, in: Lipids in Aquatic Ecosystems, edited by: Kainz, M., Brett, M. T., and Arts, M. T., Springer, New York, USA, 1–24, 2009.
Hoffman, E. J. and Duce, R. A.: Factors influencing the organic carbon content of marine aerosols: A laboratory study, J. Geophys. Res., 81, 3667–3670, https://doi.org/10.1029/JC081i021p03667, 1976.
Kanji, Z. A., Ladino, L. A., Wex, H., Boose, Y., Burkert-Kohn, M., Cziczo, D. J., and Krämer, M.: Overview of Ice Nucleating Particles, Meteorological Monographs, 58, 1-33, https://doi.org/10.1175/amsmonographs-d-16-0006.1, 2017.
Kattner, G.: Lipid composition of Calanus finmarchicus from the north sea and the arctic. A comparative study, Comp. Biochem. Physiol., 94, 185–188, https://doi.org/10.1016/0305-0491(89)90031-X, 1989.
Kawamura, K., Ishimura, Y., and Yamazaki, K.: Four years' observations of terrestrial lipid class compounds in marine aerosols from the western North Pacific, Global Biogeochem. Cy., 17, 1003, https://doi.org/10.1029/2001GB001810, 2003.
Kelly, C. P., Cramer, C. J., and Truhlar, D. G.: Predicting Adsorption Coefficients at Air-Water Interfaces Using Universal Solvation and Surface Area Models, J. Phys. Chem. B, 108, 12882–12897, https://doi.org/10.1021/jp037210t, 2004.
Khozin-Goldberg, I.: Lipid Metabolism in Microalgae, in: The Physiology of Microalgae, edited by: Borowitzka, M. A., Beardall, J., and Raven, J. A., Springer International Publishing, Switzerland 2016, 413–484, 2016.
Longhurst, A. R.: The Atlantic Ocean, in: Ecological Geography of the Sea (Second Edition), edited by: Longhurst, A. R., Academic Press, Burlington, USA, 131–273, 2007.
Marić, D., Frka, S., Godrijan, J., Tomažić, I., Penezić, A., Djakovac, T., Vojvodić, V., Precali, R., and Gašparović, B.: Organic matter production during late summer–winter period in a temperate sea, Cont. Shelf Res., 55, 52–65, https://doi.org/10.1016/j.csr.2013.01.008, 2013.
Marie, D., Shi, X. L., Rigaut-Jalabert, F., and Vaulot, D.: Use of flow cytometric sorting to better assess the diversity of small photosynthetic eukaryotes in the English Channel, FEMS Microbiol. Ecol., 72, 165–178, https://doi.org/10.1111/j.1574-6941.2010.00842.x, 2010.
Marty, J. C., Saliot, A., Buat-Ménard, P., Chesselet, R., and Hunter, K. A.: Relationship between the lipid compositions of marine aerosols, the sea surface microlayer, and subsurface water, J. Geophys. Res.-Oceans, 84, 5707–5716, https://doi.org/10.1029/JC084iC09p05707, 1979.
Michaud, J. M., Thompson, L. R., Kaul, D., Espinoza, J. L., Richter, R. A., Xu, Z. Z., Lee, C., Pham, K. M., Beall, C. M., Malfatti, F., Azam, F., Knight, R., Burkart, M. D., Dupont, C. L., and Prather, K. A.: Taxon-specific aerosolization of bacteria and viruses in an experimental ocean-atmosphere mesocosm, Nat. Commun., 9, 2017, https://doi.org/10.1038/s41467-018-04409-z, 2018.
Mochida, M., Kitamori, Y., Kawamura, K., Nojiri, Y., and Suzuki, K.: Fatty acids in the marine atmosphere: Factors governing their concentrations and evaluation of organic films on sea-salt particles, J. Geophys. Res.-Atmos., 107, AAC 1-1–AAC 1-10, https://doi.org/10.1029/2001JD001278, 2002.
Müller, K., Lehmann, S., van Pinxteren, D., Gnauk, T., Niedermeier, N., Wiedensohler, A., and Herrmann, H.: Particle characterization at the Cape Verde atmospheric observatory during the 2007 RHaMBLe intensive, Atmos. Chem. Phys., 10, 2709–2721, https://doi.org/10.5194/acp-10-2709-2010, 2010.
Nguyen, Q. T., Kjær, K. H., Kling, K. I., Boesen, T., and Bilde, M.: Impact of fatty acid coating on the CCN activity of sea salt particles, Tellus B, 69, 1304064, https://doi.org/10.1080/16000889.2017.1304064, 2017.
Palaiomylitou, M. A., Kalimanis, A., Koukkou, A. I., Drainas, C., Anastassopoulos, E., Panopoulos, N. J., Ekateriniadou, L. V., and Kyriakidis, D. A.: Phospholipid Analysis and Fractional Reconstitution of the Ice Nucleation Protein Activity Purified fromEscherichia coli Overexpressing the in a Z Gene of Pseudomonas syringae, Cryobiology, 37, 67–76, https://doi.org/10.1006/cryo.1998.2102, 1998.
Parrish, C. C.: Lipids in Marine Ecosystems, International Scholarly Research Notices, 2013, 604045, https://doi.org/10.5402/2013/604045, 2013.
Parrish, C. C., Wangersky, P. J., Delmas, R. P., and Ackman, R. G.: Iatroscan-measured profiles of dissolved and particulate marine lipid classes over the Scotian Slope and in Bedford Basin, Mar. Chem., 23, 1–15, https://doi.org/10.1016/0304-4203(88)90019-9, 1988.
Peña-Izquierdo, J., Pelegrí, J. L., Pastor, M. V., Castellanos, P., Emelianov, M., Gasser, M., Salvador, J., and Vázquez-Domínguez, E.: The continental slope current system between Cape Verde and the Canary Islands, Sci MAr., 76, 65–78, https://doi.org/10.3989/scimar.03607.18C, 2012.
Perezgonzalez, J. D.: Fisher, Neyman-Pearson or NHST? A tutorial for teaching data testing, Front. Psychol., 6, 223–223, https://doi.org/10.3389/fpsyg.2015.00223, 2015.
Quinn, P. K., Collins, D. B., Grassian, V. H., Prather, K. A., and Bates, T. S.: Chemistry and Related Properties of Freshly Emitted Sea Spray Aerosol, Chem. Rev., 115, 4383–4399, https://doi.org/10.1021/cr500713g, 2015.
Rastelli, E., Corinaldesi, C., Dell'Anno, A., Lo Martire, M., Greco, S., Cristina Facchini, M., Rinaldi, M., O'Dowd, C., Ceburnis, D., and Danovaro, R.: Transfer of labile organic matter and microbes from the ocean surface to the marine aerosol: an experimental approach, Sci. Rep., 7, 11475, https://doi.org/10.1038/s41598-017-10563-z, 2017.
R-Core-Team: A Language and Environment for Statistical Computing, R Foundation for Statistical Computing, Vienna, Austria, 2018.
Reinthaler, T., Sintes, E., and Herndl, G. J.: Dissolved organic matter and bacterial production and respiration in the sea-surface microlayer of the open Atlantic and the western Mediterranean Sea, Limnol. Oceanogr., 53, 122–136, https://doi.org/10.4319/lo.2008.53.1.0122, 2008.
Rinaldi, M., Fuzzi, S., Decesari, S., Marullo, S., Santoleri, R., Provenzale, A., von Hardenberg, J., Ceburnis, D., Vaishya, A., O'Dowd, C. D., and Facchini, M. C.: Is chlorophyll-a the best surrogate for organic matter enrichment in submicron primary marine aerosol?, J. Geophys. Res.-Atmos., 118, 4964–4973, https://doi.org/10.1002/jgrd.50417, 2013.
Robinson, T.-B., Wurl, O., Bahlmann, E., Jürgens, K., and Stolle, C.: Rising bubbles enhance the gelatinous nature of the air-sea interface, Limnol. Oceanogr., 64, 2358–2372, https://doi.org/10.1002/lno.11188, 2019.
Russell, L. M., Hawkins, L. N., Frossard, A. A., Quinn, P. K., and Bates, T. S.: Carbohydrate-like composition of submicron atmospheric particles and their production from ocean bubble bursting, P. Natl. Acad. Sci. USA, 107, 6652–6657, https://doi.org/10.1073/pnas.0908905107, 2010.
Šantl-Temkiv, T., Lange, R., Beddows, D., Rauter, U., Pilgaard, S., Dall'Osto, M., Gunde-Cimerman, N., Massling, A., and Wex, H.: Biogenic Sources of Ice Nucleating Particles at the High Arctic Site Villum Research Station, Environ. Sci. Technol., 53, 10580–10590, https://doi.org/10.1021/acs.est.9b00991, 2019.
Schmitt-Kopplin, P., Liger-Belair, G., Koch, B. P., Flerus, R., Kattner, G., Harir, M., Kanawati, B., Lucio, M., Tziotis, D., Hertkorn, N., and Gebefügi, I.: Dissolved organic matter in sea spray: a transfer study from marine surface water to aerosols, Biogeosciences, 9, 1571–1582, https://doi.org/10.5194/bg-9-1571-2012, 2012.
Scholz-Böttcher, B., Ahlf, S., Vázquez-Gutiérrez, F., and Rullkötter, J.: Natural vs. anthropogenic sources of hydrocarbons as revealed through biomarker analysis: A case study in the southern Gulf of Mexico, B. Soc. Geol. Mex., 61, 47–56, https://doi.org/10.18268/BSGM2009v61n1a5, 2009.
Simoneit, B. R. T. and Mazurek, M. A.: Organic matter of the troposphere – II. Natural background of biogenic lipid matter in aerosols over the rural western united states, Atmos. Environ., 16, 2139–2159, https://doi.org/10.1016/0004-6981(82)90284-0, 1982.
Stillwell, W.: Membrane Polar Lipids, in: An Introduction to Biological Membranes (Second Edition), edited by: Stillwell, W., Elsevier, San Diego, USA, 63–87, 2016.
Stolle, C., Ribas-Ribas, M., Badewien, T. H., Barnes, J., Carpenter, L. J., Chance, R., Damgaard, L. R., Quesada, A. M. D., Engel, A., Frka, S., Galgani, L., Gašparović, B., Gerriets, M., Mustaffa, N. I. H., Herrmann, H., Kallajoki, L., Pereira, R., Radach, F., Revsbech, N. P., Rickard, P., Saint, A., Salter, M., Striebel, M., Triesch, N., Uher, G., Upstill-Goddard, R. C., Pinxteren, M. V., Zäncker, B., Zieger, P., and Wurl, O.: The MILAN campaign: Studying diel light effects on the air-sea interface, B. Am. Meteorol. Soc., 101, E146–E166, https://doi.org/10.1175/bams-d-17-0329.1, 2019.
Tervahattu, H., Juhanoja, J., and Kupiainen, K.: Identification of an organic coating on marine aerosol particles by TOF-SIMS, J. Geophys. Res.-Atmos., 107, https://doi.org/10.1029/2001jd001403, 2002.
Triesch, N., van Pinxteren, M., Engel, A., and Herrmann, H.: Concerted measurements of free amino acids at the Cabo Verde islands: high enrichments in submicron sea spray aerosol particles and cloud droplets, Atmos. Chem. Phys., 21, 163–181, https://doi.org/10.5194/acp-21-163-2021, 2021.
van Pinxteren, M., Barthel, S., Fomba, K. W., Muller, K., von Tumpling, W., and Herrmann, H.: The influence of environmental drivers on the enrichment of organic carbon in the sea surface microlayer and in submicron aerosol particles – measurements from the Atlantic Ocean, Elementa-Sci. Anthrop., 5, 35, https://doi.org/10.1525/elementa.225, 2017.
van Pinxteren, M., Fomba, K. W., van Pinxteren, D., Triesch, N., Hoffmann, E. H., Cree, C. H. L., Fitzsimons, M. F., von Tümpling, W., and Herrmann, H.: Aliphatic amines at the Cape Verde Atmospheric Observatory: Abundance, origins and sea-air fluxes, Atmos. Environ., 203, 183–195, https://doi.org/10.1016/j.atmosenv.2019.02.011, 2019.
van Pinxteren, M., Fomba, K. W., Triesch, N., Stolle, C., Wurl, O., Bahlmann, E., Gong, X., Voigtländer, J., Wex, H., Robinson, T.-B., Barthel, S., Zeppenfeld, S., Hoffmann, E. H., Roveretto, M., Li, C., Grosselin, B., Daële, V., Senf, F., van Pinxteren, D., Manzi, M., Zabalegui, N., Frka, S., Gašparović, B., Pereira, R., Li, T., Wen, L., Li, J., Zhu, C., Chen, H., Chen, J., Fiedler, B., von Tümpling, W., Read, K. A., Punjabi, S., Lewis, A. C., Hopkins, J. R., Carpenter, L. J., Peeken, I., Rixen, T., Schulz-Bull, D., Monge, M. E., Mellouki, A., George, C., Stratmann, F., and Herrmann, H.: Marine organic matter in the remote environment of the Cape Verde islands – an introduction and overview to the MarParCloud campaign, Atmos. Chem. Phys., 20, 6921–6951, https://doi.org/10.5194/acp-20-6921-2020, 2020.
van Wambeke, F., Goutx, M., Striby, L., Sempéré, R., and Vidussi, F.: Bacterial dynamics during the transition from spring bloom to oligotrophy in the northwestern Mediterranean Sea: Relationships with particulate detritus and dissolved organic matter, Mar. Ecol. Prog. Ser., 212, 89–105, https://doi.org/10.3354/meps212089, 2001.
Wakeham, S. G., Hedges, J. I., Lee, C., Peterson, M. L., and Hernes, P. J.: Compositions and transport of lipid biomarkers through the water column and surficial sediments of the equatorial Pacific Ocean, Deep Sea Res., 44, 2131–2162, https://doi.org/10.1016/S0967-0645(97)00035-0, 1997.
Wickham, H.: tidyverse: Easily Install and Load the “Tidyverse”, R Package version 1.2.1, 2017.
Wilson, T. W., Ladino, L. A., Alpert, P. A., Breckels, M. N., Brooks, I. M., Browse, J., Burrows, S. M., Carslaw, K. S., Huffman, J. A., Judd, C., Kilthau, W. P., Mason, R. H., McFiggans, G., Miller, L. A., Najera, J. J., Polishchuk, E., Rae, S., Schiller, C. L., Si, M., Temprado, J. V., Whale, T. F., Wong, J. P. S., Wurl, O., Yakobi-Hancock, J. D., Abbatt, J. P. D., Aller, J. Y., Bertram, A. K., Knopf, D. A., and Murray, B. J.: A marine biogenic source of atmospheric ice-nucleating particles, Nature, 525, 234–238, https://doi.org/10.1038/nature14986, 2015.
Wurl, O. and Holmes, M.: The gelatinous nature of the sea-surface microlayer, Mar. Chem., 110, 89–97, https://doi.org/10.1016/j.marchem.2008.02.009, 2008.
Wurl, O., Wurl, E., Miller, L., Johnson, K., and Vagle, S.: Formation and global distribution of sea-surface microlayers, Biogeosciences, 8, 121–135, https://doi.org/10.5194/bg-8-121-2011, 2011.
Yoshimura, K., Ogawa, T., and Hama, T.: Degradation and dissolution properties of photosynthetically-produced phytoplankton lipid materials in early diagenesis, Mar. Chem., 114, 11–18, https://doi.org/10.1016/j.marchem.2009.03.002, 2009.