the Creative Commons Attribution 4.0 License.
the Creative Commons Attribution 4.0 License.
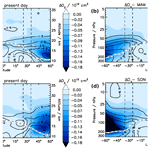
Seasonal impact of biogenic very short-lived bromocarbons on lowermost stratospheric ozone between 60° N and 60° S during the 21st century
Javier Alejandro Barrera
Rafael Pedro Fernandez
Fernando Iglesias-Suarez
Carlos Alberto Cuevas
Jean-Francois Lamarque
Biogenic very short-lived bromocarbons (VSLBr) currently represent ∼25 % of the total stratospheric bromine loading. Owing to their much shorter lifetime compared to anthropogenic long-lived bromine (e.g. halons) and chlorine (e.g. chlorofluorocarbons), the impact of VSLBr on ozone peaks in the lowermost stratosphere, which is a key climatic and radiative atmospheric region. Here we present a modelling study of the evolution of stratospheric ozone and its chemical loss within the tropics and at mid-latitudes during the 21st century. Two different experiments are explored: considering and neglecting the additional stratospheric injection of 5 ppt biogenic bromine naturally released from the ocean. Our analysis shows that the inclusion of VSLBr results in a realistic stratospheric bromine loading and improves the agreement between the model and satellite observations of the total ozone column (TOC) for the 1980–2015 period at mid-latitudes. We show that the overall ozone response to VSLBr at mid-latitudes follows the stratospheric evolution of long-lived inorganic chlorine and bromine throughout the 21st century. Additional ozone loss due to VSLBr is maximized during the present-day period (1990–2010), with TOC differences of −8 DU (−3 %) and −5.5 DU (−2 %) for the Southern Hemisphere and Northern Hemisphere mid-latitudes (SH-MLs and NH-MLs), respectively. Moreover, the projected TOC differences at the end of the 21st century are ∼50 % lower than the values found for the present-day period.
We find that seasonal VSLBr impact on lowermost stratospheric ozone at mid-latitude is influenced by the seasonality of the heterogeneous inorganic-chlorine reactivation processes on ice crystals. Indeed, due to the more efficient reactivation of chlorine reservoirs (mainly ClONO2 and HCl) within the colder SH-ML lowermost stratosphere, the seasonal VSLBr impact shows a small but persistent hemispheric asymmetry through the whole modelled period. Our results indicate that, although the overall VSLBr-driven ozone destruction is greatest during spring, the halogen-mediated (Halogx-Loss) ozone loss cycle in the mid-latitude lowermost stratosphere during winter is comparatively more efficient than the HOx cycle with respect to other seasons. Indeed, when VSLBr are considered, Halogx-Loss dominates wintertime lowermost stratospheric ozone loss at SH-MLs between 1985 and 2020, with a contribution of inter-halogen ClOx–BrOx cycles to Halogx-Loss of ∼50 %.
Within the tropics, a small ( DU) and relatively constant ( %) ozone depletion mediated by VSLBr is closely related to their fixed emissions throughout the modelled period. By including the VSLBr sources, the seasonal Halogx-Loss contribution to lowermost stratospheric ozone loss is practically dominated by the BrOx cycle, reflecting the low sensitivity of very short-lived (VSL) bromine to background halogen abundances to drive tropical stratospheric ozone depletion. We conclude that the link between biogenic bromine sources and seasonal changes in heterogeneous chlorine reactivation is a key feature for future projections of mid-latitude lowermost stratospheric ozone during the 21st century.
- Article
(2149 KB) - Full-text XML
-
Supplement
(2423 KB) - BibTeX
- EndNote
The role of bromine in stratospheric ozone depletion has been discussed in several studies (Wofsy et al., 1975; Prather and Watson, 1990; Daniel et al., 1999; Dvortsov et al., 1999; Solomon, 1999). Although bromine is much less abundant than chlorine in the atmosphere, it is known to deplete stratospheric ozone 45 to 69 times more efficiently on a per atom basis (Daniel et al., 1999; Sinnhuber et al., 2009). Moreover, the ozone depletion efficiency of active bromine (Br and BrO) is strongly related to the available amount of activated chlorine (mainly Cl and ClO radicals) in the atmosphere (McElroy et al., 1986; Solomon et al., 1999, and references therein) as well as to enhanced sulfate aerosol loading via heterogeneous reactions (Salawitch et al., 2005). Consequently, even low concentrations of bromine have a relatively large impact on stratospheric ozone. In addition to anthropogenic long-lived chlorine (LLCl) and bromine (LLBr) substances such as chlorofluorocarbons (CFCs), chlorocarbons, methyl bromide, and bromofluorocarbons (halon fire suppressants), other substances with photochemical lifetimes shorter than 6 months, often referred to as very short-lived (VSL) substances, have the potential to transport a significant amount of reactive halogens into the stratosphere. Owing to their short lifetimes, the impact of VSL substances on stratospheric ozone peaks in the lowermost stratosphere (Salawitch et al., 2005; Feng et al., 2007; Sinnhuber et al., 2009; Sinnhuber and Meul, 2015; Yang et al., 2014; Hossaini et al., 2015a; Falk et al., 2017), which is an important atmospheric region because surface temperature and climate are most sensitive to ozone perturbations (Riese et al., 2012; Hossaini et al., 2015b; Iglesias-Suarez et al., 2018). In fact, Hossaini et al. (2015b) found that very short-lived bromocarbons (VSLBr) exert an ozone radiative effect (normalized by halogen content) that is 3.6 times larger than that arising from long-lived substances.
VSLBr are produced in the ocean via the metabolism of marine organisms such as phytoplankton and macroalgae (e.g. Carpenter et al., 2007; Quack et al., 2007; Leedham Elvidge et al., 2015), even in sea ice (e.g. Abrahamsson et al., 2018). Due to their high volatility, they are transferred into the marine boundary layer through air–sea exchange (Carpenter and Liss, 2000; Quack and Wallace, 2003). The most abundant VSLBr released to the atmosphere are bromoform (CHBr3) and dibromomethane (methylene dibromide, CH2Br2), followed by a minor (but non-negligible) contribution of inter-halogen species (bromochloromethane, CH2BrCl; dibromochloromethane, CHBr2Cl; and bromodichloromethane, CHBrCl2). Altogether, VSLBr contribute ∼5 (3–7) ppt to stratospheric bromine, which accounted for about 25 % of total stratospheric bromine in 2016 (WMO, 2018). Moreover, this additional input of bromine is required to reconcile current stratospheric bromine trends (Salawitch et al., 2010; WMO, 2018).
Production of anthropogenic LLCl and LLBr substances has been restricted by the Montreal Protocol and its subsequent amendments and adjustments (Solomon, 1999; WMO, 2018). After application, the stratospheric LLCl and LLBr load peaked at the end of the 20th century and has been decreasing at a rate that depends on their respective atmospheric lifetimes. This implies, for example, that hydrogen chloride (HCl) shows a long-term decrease at a rate of about 0.5 % yr−1 in the middle stratosphere between 60∘ N and 60∘ S, while total stratospheric bromine decreased at a rate of about 0.75 % yr−1 from 2004 to 2014 (WMO, 2018). Accordingly, stratospheric ozone is expected to recover from the effects of anthropogenic halogen-induced loss on a similar timescale, which is already detectable in the Antarctic and the upper stratosphere (e.g. Solomon et al., 2016a; Chipperfield et al., 2017; Dhomse et al., 2018; Strahan and Douglass, 2008, and references therein). Hossaini et al. (2015a) quantified the stratospheric injection of organic and inorganic chlorine from anthropogenic VSLCl sources such as chloroform (CHCl3), dichloromethane (CH2Cl2), tetrachloroethene (C2Cl4), trichloroethene (C2HCl3), and 1,2-dichloroethane (CH2ClCH2Cl), which are not controlled by the Montreal Protocol. From their results, the total stratospheric chlorine load from VSLCl inferred for 2013 is 123 ppt, with a stratospheric injection dominated by source gases (∼83 %). Moreover, the stratospheric injection of VSLCl increased by ∼52 % between 2005 and 2013, mainly due to recent and ongoing growth in anthropogenic CH2Cl2 emissions. In fact, Hossaini et al. (2017) showed that the impact of CH2Cl2 on stratospheric ozone has increased markedly in recent years, and if these increases continue into the future, the recovery of the Earth's ozone layer could be delayed even further, offsetting some of the gains achieved by the Montreal Protocol.
The total stratospheric chlorine and bromine budgets derived for 2016 were 3.29 ppb Cl and 19.60 ppt Br, respectively (WMO, 2018), and are expected to return to their 1980 values, an arbitrary reference date before the discovery of the Antarctic ozone hole, around the middle of this century (Dhomse et al., 2018). Although we still lack scientific consensus with respect to the future evolution of VSLBr oceanic source strength and stratospheric injection (WMO, 2014; Lennartz et al., 2015; Ziska et al., 2017), the continuous decrease in LLBr leads to an increase in the relative VSLBr contribution to total stratospheric bromine into the future. Thus, understanding the role of natural VSLBr sources is a key issue for chemistry–climate projections.
The impact of additional bromine is closely related to the heterogeneous chemistry of chlorine (Solomon, 1999; Salawitch et al., 2005; Müller, 2012). The influence of temperature and water vapour in the heterogeneous conversion processes of inorganic-chlorine reservoirs (mostly HCl and ClONO2) into active radicals on sulfate aerosols has been reviewed elsewhere (e.g. Anderson et al., 2012, 2017; Drdla and Müller, 2012; Anderson and Clapp, 2018). In particular, Drdla and Müller (2012) highlighted that an increase in water vapour above background values would allow chlorine activation at higher temperatures than those observed in polar regions, which led to the hypothesis that chlorine reactivation and subsequent ozone loss could occur in the lower stratosphere at mid-latitudes during summer (Robrecht et al., 2019). Indeed, the spatial and seasonal distributions of chlorine monoxide and chlorine nitrate in the monsoon regions provide indicators of heterogeneous chlorine processing in the tropical and subtropical lowermost stratosphere of the Northern Hemisphere (Solomon et al., 2016b). Moreover, heterogeneous chorine reactivation has also been observed on ice particles in cirrus clouds located near the tropopause (Borrman et al., 1996, 1997; Solomon et al., 1997; Bregman et al., 2002; Thornton et al., 2003). The occurrence of cirrus clouds was reported in the lowermost stratosphere of northern high-mid-latitudes (40–75∘ N; Spang et al., 2015), with summertime clouds located up to ∼3 km (or 40–50 K of potential temperature) above the tropopause (Dessler, 2009). Furthermore, von Hobe et al. (2011) suggested that cirrus ice particles and/or liquid aerosol at low temperatures may promote a significant activation of heterogeneous chlorine in the tropical upper troposphere and lower stratosphere. Finally, satellite observations indicate that chlorine activation also occurs in the Antarctic and Arctic sub-vortex regions, where processed air dispersing from the decaying vortex in spring induces rapid changes in extra-vortex trace gas abundances (Santee et al., 2011).
Previous chemistry–climate modelling studies considering VSLBr chemistry have mainly focused on improving the model vs. observed mid-latitude ozone trends at the end of the 20th century (Feng et al., 2007; Sinnhuber et al., 2009). More recently, Sinnhuber and Meul (2015) have shown that the model simulations including VSLBr resulted in a much greater ozone decrease in the lowermost stratosphere during the 1979–1995 period and a faster ozone increase during the 1996–2005 period, which is in better agreement with observed ozone trends. Moreover, Falk et al. (2017) have reported that the projected additional VSLBr impact on ozone at the end of the 21st century is reduced compared to present day. These results are in agreement with those of Yang et al. (2014), who performed time slice simulations to address the sensitivity of stratospheric ozone to a speculative doubling of VSLBr sources under different LLCl scenarios. However, neither of the previous studies had distinguished the contribution to ozone depletion – including its seasonal variability – that arises from VSLBr with respect to LLCl and LLBr throughout the 21st century.
In this work, using the CAM-chem model, we present a coupled chemistry–climate modelling study from 1960 to 2100 with and without the contribution from VSLBr sources. We focus on natural VSLBr-driven impacts on the temporal evolution of stratospheric ozone and the total ozone column (TOC) in the tropics and at mid-latitudes, distinguishing both the long-term seasonal change and the resulting hemispheric asymmetries. Additionally, we present a timeline assessment of the individual contribution from anthropogenic (LLCl and LLBr) and biogenic (VSLBr) sources to stratospheric halogen loading throughout the 21st century while also recognizing the VSLBr contribution to overall halogen-catalysed ozone loss in the lowermost stratosphere. The layout of the paper is as follows: Sect. 2 describes the main characteristics and configuration of the CAM-chem model as well as the observation data sets that are used for the evaluation of the CAM-chem performance. Section 3 presents a quantitative comparison between the model and observations of stratospheric chlorine and bromine loading (Sect. 3.1) and the TOC (Sect. 3.2) as well as discusses long-term seasonal impacts mediated by VSLBr on the TOC (Sect. 3.2) and the lowermost stratospheric ozone budget (Sect. 3.3). Changes in the seasonal evolution of halogen-driven ozone loss rates due to VSLBr are shown in Sect. 3.4. The final concluding remarks are summarized in Sect. 4.
The Community Earth System Model (CESM1) with the Community Atmospheric Model including interactive chemistry (CAM4-chem version; Lamarque et al., 2012; Tilmes et al., 2016) has been used to explore VSLBr-driven stratospheric ozone loss in three latitude bands: Southern Hemisphere mid-latitudes (SH-MLs; 60–35∘ S), tropics (25∘ N–25∘ S), and Northern Hemisphere mid-latitudes (NH-MLs; 35–60∘ N). The model set-up follows the Chemistry–Climate Model Initiative (CCMI) REFC2 configuration described in detail by Tilmes et al. (2016), with the exception that in this work we consider a full halogen chemistry mechanism and oceanic emission – seasonally dependent and geographically distributed – of six bromocarbons (VSLBr = CHBr3, CH2Br2, CH2BrCl, CHBrCl2, CHBr2Cl, and CH2IBr) from the Ordóñez et al. (2012) emission inventory. This emission inventory is based on a monthly varying satellite chl a climatology, which allows the introduction of a complete seasonal cycle to the emission strength and spatial distribution of oceanic bromocarbons. A full description of the VSLBr mechanism implemented in CAM-chem – including both biogenic and anthropogenic sources, heterogeneous recycling reactions, dry and wet deposition, convective uplift, and large-scale transport – has been given elsewhere (Ordóñez et al., 2012; Fernandez et al., 2014). Monthly and seasonally varying lower boundary conditions were considered for long-lived chlorine (LLCl = CH3Cl, CH3CCl3, CCl4, CFC-11, CFC-12, CFC-113, HCFC-22, CFC-114, CFC-115, HCFC-141b, and HCFC-142b) and long-lived bromine (LLBr = CH3Br, H-1301, H-1211, H-1202, and H-2402) following the A1 halogenated ozone-depleting substance emissions scenario (WMO, 2011). The surface concentrations of CO2, CH4, H2, and N2O were specified following the moderate Representation Concentration Pathway 6.0 (RCP6.0) scenario (Meinshausen et al., 2011; Eyring et al., 2013).
The CAM-chem configuration used in this work extends from the surface to approximately 40 km (3.5 hPa in the upper stratosphere), with 26 vertical levels, and includes a horizontal resolution of 1.9∘ latitude by 2.5∘ longitude. The number of stratospheric levels changes depending on the latitudinal region: within the tropics, there are eight levels above the tropopause (∼100 hPa), with a mean thickness of 1.25 km (15.5 hPa) for the lower stratospheric levels and 5.2 km (3.8 hPa) between the two highest levels, while for the mid-latitudes, the tropopause is located at approximately ∼200 hPa, and the stratosphere contains up to 12 levels. To ensure a consistent dynamical description of the stratosphere within the relatively low model top of CAM-chem (i.e. gravity wave dragging and the Brewer–Dobson circulation), the integrated momentum that would have been deposited above the model top is specified by an upper boundary condition (Lamarque et al., 2008). A similar procedure is applied to the altitude-dependent photolysis rate computations, which include an upper boundary condition that considers the ozone column fraction prevailing above the model top. The CAM4-chem version used in this work includes a non-orographic gravity wave scheme based on the inertia–gravity wave parameterization and an observation-based implementation of stratospheric aerosol and surface area density. The quasi-biennial oscillation is imposed in the model by relaxing equatorial zonal winds between 90 and 3 hPa to the observed interannual variability (see Tilmes et al., 2016, for more details).
Equivalent CAM-chem configurations, such as the one implemented in this work, have been used for the CCMVal-2 (Chemistry–Climate Model Validation 2) and CMIP5 (Coupled Model Intercomparison Project, Phase 5) activities in order to represent trends in the ozone evolution and to estimate the return dates, which lie in the middle of the multi-model range (Eyring et al., 2010, 2013). Moreover, our model configuration uses a fully coupled Earth system model approach, i.e. the ocean and sea ice are explicitly computed (Neale et al., 2013). This implies that instead of isolating the chemical impact mediated by VSLBr on the climatological ozone budget (as would be the case using a specified dynamic approach), the chemical interaction between VSL bromine and ozone in the lowermost stratosphere is affected by dynamic feedbacks (i.e. temperature, radiation, etc.).
Even though the interactive ocean coupling would have allowed us to compute the evolution of VSLBr ocean source emissions throughout the modelled period, we still lack a considerable understanding of the seasonal processes that dominate VSLBr ocean emissions in both the present and the future, which is combined with a limited observation data set of VSL bromocarbons (WMO, 2014, 2018). Therefore, in this work, we forced the model with annually cycled VSLBr fluxes, replicating the Ordoñez et al. emission inventory for all years between 1950 and 2100. This procedure aims to reduce the uncertainties associated with the unknown evolution of both biogeochemical production and oceanic emissions of VSLBr into the future (Lennartz et al., 2015; Ziska et al., 2017).
Two ensembles of independent modelled experiments were performed, each with three individual simulations from 1960 to 2100, considering approximately 10 years of spin-up to allow stabilization of the stratospheric circulation. The individual simulations 001, 002, and 003 started in 1950, 1951, and 1952, respectively, with initial conditions taken from the equivalent years of the CESM1 WACCM (Whole Atmosphere Community Climate Model) 20th-century ensemble for CMIP5 (Marsh et al., 2013). The baseline simulation set-up of the first experiment considered only the halogen LLCl and LLBr contribution from anthropogenic chlorofluorocarbons, hydrochlorofluorocarbons, halons, methyl chloride, and methyl bromide, while the simulation set-up of the second experiment included the background biogenic contribution from VSLBr oceanic sources in addition to the anthropogenic sources above. The mean ensemble of each experiment (i.e. with and without the VSLBr sources) was calculated as the average of the three individual simulations. Differences between these two mean ensembles allow quantification of the overall impact of VSLBr on stratospheric ozone as well as the determination of their relative contributions to the halogen-mediated catalytic ozone-depleting families. In addition, from this analysis it is also possible to distinguish the contributions of both LLBr and VSLBr to the inorganic fraction of stratospheric bromine (), whereas the inorganic fraction of chlorine () arises only from the degradation of LLCl, which is identical for both experiments.
For the case of vertical distributions and latitudinal variations, the zonal mean of each mean ensemble was computed from the monthly output before processing the data, while a locally weighted scatter plot smoothing (LOWESS) filter with a 0.2 fraction was applied to all long-term time series to smooth the data. Most of the figures and values in the Results section include geographically averaged quantities for the present day (defined here as the 1990–2010 mean, nominally year 2000) and the end of the 21st century (defined here as the 2080–2100 mean, nominally year 2100) over the latitudinal bands defined above. To highlight seasonal changes, we computed the average for the months of December–January–February (DJF), March–April–May (MAM), June–July–August (JJA), and September–October–November (SON). Ozone loss trends due to VSLBr between 2000 and 2100 were calculated on the basis of the least-squares linear trends, while the trend errors were estimated as twice the statistical deviation stemming from the least-squares fit.
To provide a basic evaluation of the model performance, the modelled results of the TOC and the inorganic-halogen abundances were compared with the following selected observation data sets:
- i.
Microwave Limb Sounder (MLS) observations of the annual mean volume mixing ratio of HCl + ClO (Waters et al., 2006; Livesey et al., 2018), which provides a lower limit of the Cly abundance in the stratosphere from 2005 to 2015;
- ii.
Total stratospheric Bry trend reported in the latest WMO Ozone Assessment Reports (WMO, 2014, 2018), which shows changes in total Bry between 1991 and 2012 derived from stratospheric BrO observations by balloon-borne (Dorf et al., 2006) and ground-based UV–visible (at Harestua, 60∘ N, and Lauder, 45∘ S) measurements (Hendrick et al., 2007, 2008);
- iii.
The Solar Backscatter Ultraviolet (SBUV) merged total ozone column data set (MOD; Frith et al., 2014, 2017) from 1980 to 2015.
An important point to highlight about the SBUV MOD (and its uncertainties) is that it was constructed from ozone profiles measured by individual SBUV instruments and retrieved using the Version 8.6 algorithm (Bhartia et al., 2013; Frith et al., 2014, 2017). To maintain consistency over the entire time series, the individual instrument records were analysed with respect to each other, and absolute calibration adjustments were applied as needed based on comparison of radiance measurements during periods of instrument overlap (DeLand et al., 2012; Weber et al., 2018). During the overlap periods, the records of the involved SBUV instruments were combined using a simple average. Frith et al. (2014) have estimated the potential errors in the SBUV MOD through Monte Carlo model simulations, taking into account the range of offsets and drifts observed during the overlap periods between individual SBUV instruments and between SBUV and Dobson ground-based measurements. The authors also assessed the extent to which these potential errors might affect the long-term variability of ozone in a multiple-regression model. See Frith et al. (2014) for a detailed description of the data used and their associated uncertainties in the SBUV MOD.
3.1 Contribution of LLCl, LLBr, and VSLBr to stratospheric inorganic-halogen loading
The evolution (1960–2100) of the modelled annual mean abundances of inorganic chlorine (Cly) and bromine (Bry) as well as the VSLBr in the global upper stratosphere (3.5 hPa) and lower stratosphere (50 hPa) of the tropics and mid-latitudes is shown in Fig. 1. In addition, Fig. 1 includes the total Bry trends (WMO, 2014, 2018) and the MLS observations (HCl + ClO). The values of bias, normalized mean bias (NMB), and normalized mean error (NME) used to evaluate the agreement between the modelled Cly and observations for each of the regions under study are shown in Table S1 in the Supplement. Clearly, the temporal evolution of and shows a pronounced peak at the end of the 20th century and beginning of the 21st century, respectively, after which the abundance of both halogens declines. Within the A1 halogen emission scenario considered in this work, the abundance in our model returns to its past 1980 levels just before ∼2060 in the global upper stratosphere, whereas the prevailing abundance for the year 1980 is not recovered even by the end of the 21st century (see Fig. 1a). In comparison, the evolution of abundances remains constant over time, resulting in an additional and time-independent fixed contribution of ∼5 ppt total bromine injection, which leads to maximum global upper-stratosphere abundances of up to ∼20.5 ppt at the beginning of the 21st century. This is in agreement with previous studies performed only for present-day conditions (Fernandez et al., 2014). Furthermore, note that the modelled global abundances are in good agreement with the observations within reported errors, highlighting the importance of considering the additional contribution from VSLBr to determine the overall evolution of stratospheric ozone during the 21st century.
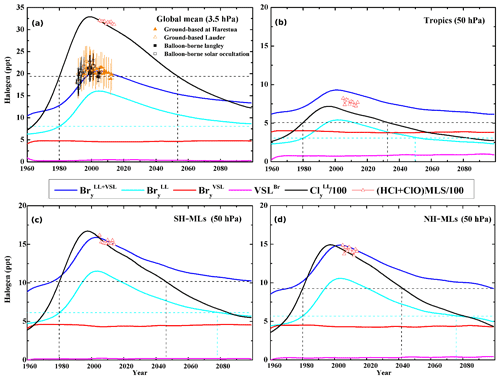
Figure 1Temporal evolution (1960–2100) of the modelled annual mean abundances of VSLBr and inorganic halogen ( and ): (a) global upper stratosphere (3.5 hPa); lower stratosphere (50 hPa) in the (b) tropics and at (c) SH-MLs and (d) NH-MLs. The abundance was split into long-lived () and very short-lived () contributions. The black squares (filled and open) and orange triangles (filled and open) of panel (a) show the total stratospheric Bry trends from 1991 to 2012 (WMO, 2014, 2018). The red triangles show the mean annual mixing ratios (HCl + ClO) from the Microwave Limb Sounder (MLS) data (Waters et al., 2006; Livesey et al., 2018) from 2005 to 2015. The dashed horizontal lines indicate the modelled and abundances for 1980. Note that both values and (HCl + ClO) MLS data were divided by 100.
In the lower stratosphere, at 50 hPa, abundances return to 1980 levels before ∼2080 and ∼2050 for the mid-latitudes and tropics, respectively, while the return date remains unaltered (see Fig. 1b–d). Furthermore, the contribution from VSLBr to mid-latitude total inorganic bromine also reaches ∼5 ppt throughout the modelled period, while in the tropics there prevails a small carbon-bonded organic fraction that has not been converted to the reactive inorganic form. Falk et al. (2017) showed an increase in VSLBr of about 0.5 ppt in the tropical lowermost stratosphere by the end of the century compared to present day under the RCP6.0 scenario and assuming constant VSLBr fluxes, following scenario five of Warwick et al. (2006). This increase in the stratospheric VSLBr attributed to enhanced vertical transport in the tropics is counteracted by a decrease in the injected into the stratosphere so that the total stratospheric VSL bromine remains unchanged between the two periods. In this work, the stratospheric injection of VSLBr and between 2000 and 2100 remains unchanged. Based on the limited observational data, it is uncertain whether any trend in the stratospheric injection of organic and inorganic VSL bromine would be expected, and thus no conclusive statement can be made. Nevertheless, our present-day stratospheric injection of VSLBr (∼2 ppt) and (∼3 ppt) is in perfect accordance with that reported in the latest WMO Ozone Assessment Report (WMO, 2018). Further studies are needed to evaluate the uncertain evolution of the stratospheric injection of organic and inorganic species from VSLBr throughout the 21st century.
The modelled inorganic-chlorine abundance shows a good agreement with the (HCl + ClO) MLS observations for the 2005–2015 period mainly in the upper stratosphere, where most chlorine has already been photochemically converted to . For example, the normal mean error of the comparison between modelled abundances and MLS observations is about 3.5 % and 19 % at 50 hPa for the mid-latitudes and tropics, respectively, while the NME is about 2 % for the global mean at 3.5 hPa (see Table S1). This relatively good agreement between the model and MLS observation occurs even without consideration of the recent contribution of anthropogenic VSLCl sources (i.e. Hossaini et al., 2015b, 2017). Moreover, the inter-annual variation in MLS observations at 50 hPa occurs mainly due to heterogeneous chlorine reactivation, which can be influenced by the polar vortex dynamics at mid-latitudes (Dhomse et al., 2018). Note that although we processed output at 120 hPa in the mid-latitude lowermost stratosphere in Sect. 3.4, we did not compare at this altitude level. This is because below 50 hPa, the fractional conversion of organic chlorine to is very small, and thus the inferred HCl + ClO MLS data have a very large uncertainty that prevents a reliable model-observation comparison (Santee et al., 2011).
The stratospheric injection remains constant during the whole modelled period, representing ∼25 % of in the global upper stratosphere for the year 2000 and increasing up to ∼40 % by the end of the 21st century. These results are in agreement with those reported by Fernandez et al. (2017). Due to the much shorter lifetimes of VSLBr with respect to LLBr, the contribution percentage is larger in the lower stratosphere. For example, the relative contribution to for the year 2000 at 50 hPa represents ∼30 % and ∼45 % for the mid-latitudes and tropics, respectively. Furthermore, at mid-latitudes, represents up to 45 % of both at the end of the 21st century and in the years prior to 1980, while in the tropics represents up to 65 % for these time periods. Consequently, these changes in the relative contribution of VSLBr to total inorganic bromine with respect to time, region, and altitude in the presence of different inorganic-chlorine abundances lead to relatively different ozone loss within those regions and altitude regimes, as described in detail below.
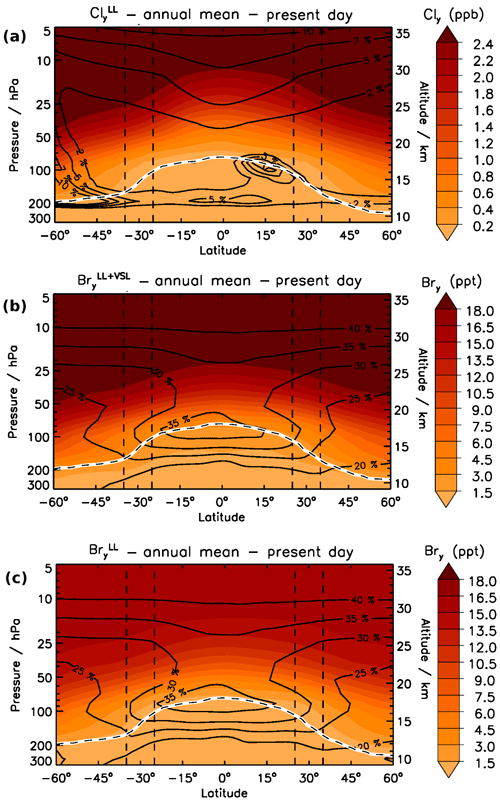
Figure 2Annual zonal mean distribution of (a) , (b) , and (c) during the present-day period. The colour scale represents volume mixing ratios (ppb or ppt), while black contour lines show the contribution percentage of ClOx to Cly and BrOx to Bry. The lower solid white line indicates the location of the tropopause (chemical definition of 150 ppb ozone level from the experiment without VSLBr sources).
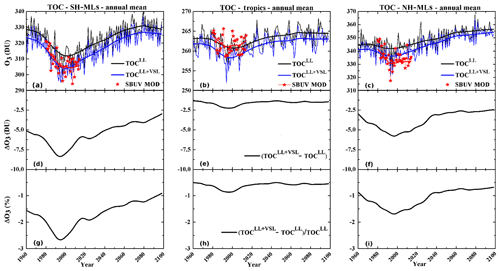
Figure 3Temporal evolution of the annual mean total ozone column (TOCLL+VSL and TOCLL) (a) at SH-MLs, (b) in the tropics, and (c) at NH-MLs as well as the corresponding absolute (DU; d–f) and relative (%; g–i) TOC difference (ΔTOC). TOC values of the mean ensemble (thin lines) and the smoothed time series via LOWESS filtering (0.2 fractions; thick lines) are shown in blue for TOCLL+VSL and black for TOCLL. The red lines and symbols show the observations from the Solar Backscatter Ultraviolet (SBUV) merged total ozone column data set (Frith et al., 2014, 2017) within the same spatial and temporal mask as the model output.
Figure 2 shows the annual zonal mean distribution of inorganic-chlorine and inorganic-bromine abundances as well as the corresponding ClOx∕Cly and BrOx∕Bry percentage ratios (black contour lines) for the present day. Note that the ClOx∕Cly and BrOx∕Bry ratios reflect the changes in the contribution of the active-chlorine () and active-bromine () fractions relative to their total inorganic abundances. Equivalent results are presented for the end of the 21st century in the Supplement (see Fig. S1). In the lowermost stratosphere, the available inorganic fraction of both chlorine and bromine rapidly increases with altitude as halogen atoms are released by the photolysis and oxidation from all LLCl, LLBr, and VSLBr species. In contrast, within the upper stratosphere no major changes in inorganic-halogen abundances are observed since the conversion from their organic sources is nearly complete. Although our model shows a symmetric hemispheric distribution in stratospheric Cly and Bry abundances, there is a marked difference in the ClOx∕Cly ratio. In fact, the experiments capture a local increase in the ClOx∕Cly ratio at 15∘ N and ∼100 hPa as a result of a large increase in heterogeneous reactivation of Cly species due to enhanced vertical transport during the Indian summer monsoon (Solomon et al., 2016b). Along the same lines, the higher ClOx∕Cly values modelled in the lowermost stratosphere at SH-MLs compared to NH-MLs are mainly due to the enhancement in the heterogeneous chlorine reactivation processes on ice crystals (i.e. HCl and ClONO2 reactivation; Santee et al., 2011; Solomon, 1999, and references therein), influenced mainly by the proximity of the Antarctic polar vortex edge and the lower temperatures prevailing at high- and mid-latitudes of the Southern Hemisphere (see Fig. S2). Additionally, the favourable conditions for isentropic exchange of young subtropical air with extremely old winter polar vortex air masses at SH-MLs (Spang et al., 2015; Rolf et al., 2015) could drive additional heterogeneous chlorine reactivation and thus enhance hemispheric asymmetry. Moreover, the increase in the ClOx abundance and ClOx∕Cly ratio is highest during winter and spring for both SH-MLs and NH-MLs, which is consistent with the seasonal changes of the lowermost stratospheric temperatures that affect chlorine reactivation on ice crystals (see Fig. S3). In contrast, a symmetric hemispheric distribution of the BrOx∕Bry ratio is modelled in the mid-latitude lowermost stratosphere and is associated with heterogeneous bromine reactivation on sulfate aerosols instead of ice crystals (i.e. HBr and BrONO2 reactivation; Solomon, 1999, and references therein), which show neither any hemispheric asymmetry nor seasonal changes in the reactivation processes (see Fig. S4). Thus, even when the inclusion of VSLBr sources increases the total stratospheric Bry abundance and therefore its active fraction (BrOx), the BrOx∕Bry ratio remains unchanged in the stratosphere during the whole modelled period (see Figs. 2b, c and S1b, c), highlighting that this ratio is nearly independent of the total inorganic bromine abundance.
Table 1Annual and seasonal TOC changes (ΔTOC) mediated by VSLBr at mid-latitudes and in the tropics for the present day and the end of the 21st century as well as the ΔTOC trends (% per decade) over the century.
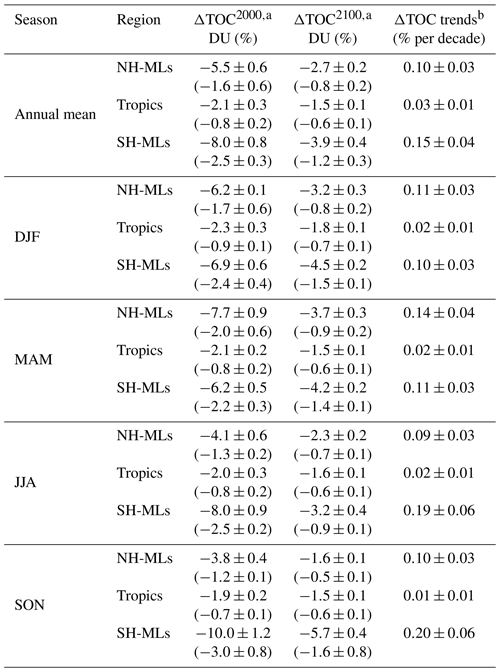
a Absolute (DU) and relative (%; in brackets) TOC changes during the present-day period (ΔTOC2000) and the end of the 21st century (ΔTOC2100) were computed considering the mean ensemble of each experiment at mid-latitudes (SH-MLs and NH-MLs) and in the tropics. Annual and seasonal ΔTOC errors were estimated from standard mean errors. b Trend errors were estimated as twice the statistical deviation stemming from the least-squares fit.
3.2 Impact of VSLBr on the seasonal evolution of the total ozone column (TOC)
The temporal evolution (1960–2100) of the modelled annual mean TOC at mid-latitudes and in the tropics, along with SBUV MOD observations, is illustrated in Fig. 3. Equivalent results for the temporal evolution of the individual simulations of each experiment are shown in Fig. S5. Table 1 shows the annual and seasonal mean values of the absolute and relative TOC differences (ΔTOC) between the experiments for the present day and the end of the 21st century as well as the ΔTOC trends (% per decade) over the century. In addition, Table S2 shows the values of bias, normalized mean bias (NMB), and normalized mean error (NME) to evaluate the agreement between the modelled TOC and observations for each of the regions under study. Based on the comparison of the TOC results over the different latitudinal bands, the following features can be described:
- i.
The constant emission of VSLBr introduces a continuous reduction in the TOC that exceeds the mean ensemble variability between the experiments (see Fig. S5). Moreover, the inclusion of VSLBr sources improves the overall agreement between the model and observations, with a reduction of 1 % and 0.9 % in NME for SH-MLs and NH-MLs, respectively (see Table S2). However, the inclusion of VSLBr sources leads to a slight increase of 0.2 % in NME for the tropics. Note that the statistical analysis presented in Table S2 does not take into account the uncertainties that may arise from the merging of the individual SBUV instruments in the SBUV MOD (see Sect. 2).
- ii.
At mid-latitudes, the additional ozone loss due to VSLBr peaks during the present-day period, coinciding with the temporal location of the minimum TOC for both experiments and reaching a ΔTOC2000 of approximately −8 DU ( %) and −5.5 DU ( %) for SH-MLs and NH-MLs, respectively (see Table 1). Moreover, VSLBr impacts on the TOC by the end of the 21st century are ∼50 % lower than the values found for the present day, which is in line with the projected ΔTOC trends of 0.15 % and 0.10 % per decade for SH-MLs and NH-MLs, respectively.
- iii.
Within the tropics, the inclusion of VSLBr leads to small impacts on the TOC compared to impacts observed at mid-latitudes. The TOC differences due to VSLBr are close to −1.5 DU ( %) during practically the entire modelled period, with a maximum ΔTOC2000 reaching −2.1 DU ( %). This is in line with a projected ΔTOC trend of 0.03 % per decade. Moreover, even though VSLBr slightly worsen the agreement between the modelled TOCLL and observations, the minimum TOCLL+VSL is shifted to ∼5 years earlier compared to TOCLL (i.e. towards where the inorganic-chlorine peak is located in the year 1995), which is in agreement with observations.
- iv.
Overall, our model results show much higher ozone depletion due to VSLBr at mid-latitudes compared to the tropics.
Our results of the mid-latitude ΔTOC due to VSLBr lie within the lower range of previous modelling studies over the 1960–2005 (Sinnhuber and Meul, 2015) and 1980–2005 (Feng et al., 2007) periods. This is expected as the detailed treatment of VSLBr sources in Sinnhuber and Meul (2015) results in an additional injection of 6 ppt, while in Feng et al. (2007), the model configurations included the direct supply of tropospheric Bry as a lower condition in addition to a VSL substance tracer. Furthermore, our results are in accord with the future projection trends in Falk et al. (2017), although their analysis on annual mean ozone loss due to VSLBr only focused on the late 21st century (2075–2100).
Figure 4 shows the temporal evolution (1960–2100) of the annual and seasonal ΔTOC between the experiments for SH-MLs, the tropics, and NH-MLs. In addition, an analysis of ΔTOC as a function of latitude for the present day and the end of the 21st century is shown in Fig. 5. The inclusion of VSLBr leads to a continuous reduction in the TOC at all latitudes, with a larger ozone destruction efficiency moving from the tropics to the high latitudes. Moreover, note that when comparing the seasonal relative ΔTOC between both periods, a statistically significant difference (here defined as p<0.05) is only observed at mid-latitudes, as shown by the horizontal lines at the bottom of Fig. 5d. This highlights that the VSLBr-driven ozone depletion efficiency changes significantly over the century even though the contribution from VSLBr to bromine stratospheric injection remains constant. Since gas-phase and heterogeneous inter-halogen reactions involving both bromine and chlorine play a fundamental role in stratospheric ozone loss (McElroy et al., 1986; Solomon, 1999; Salawitch et al., 2005), the VSL bromine efficiency in ozone depletion is primarily linked to the background inorganic-halogen abundance, which shows a continuous decline over the course of the 21st century due to the A1 halogen emission scenario considered in this work. This is in line with the findings of Yang et al. (2014). Therefore, an additional stratospheric chlorine and bromine load from natural or anthropogenic substances not regulated by the Montreal Protocol will induce an increase in the VSLBr impacts modelled in this work on projected ozone loss trends throughout the current century.
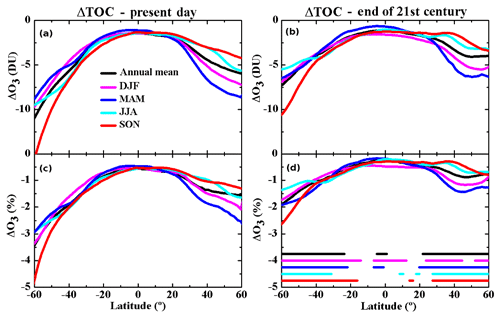
Figure 5Latitude distributions of total ozone column difference (ΔTOC) between the experiments. Panels (a) and (b) show the annual and seasonal mean absolute ΔTOC (DU) for the present day and the end of 21st century, respectively, while panels (c) and (d) show the corresponding relative ΔTOC (%). The corresponding horizontal lines shown in panel (d) indicate where the relative ΔTOC values between the present day and the end of the 21st century are statistically significant with a 95 % confidence interval using a two-tailed Student's t test.
The largest mid-latitude TOC differences between experiments occur during spring, when the maximum seasonal TOC levels are found, followed by the winter. The maximum springtime ΔTOC2000 reaches −10 DU ( %) and −7.7 DU ( %) for SH-MLs and NH-MLs, respectively (see Table 1 and Fig. 4). In contrast, during summer and autumn, the maximum ΔTOC2000 values remain below −6.8 ( %) and −4 DU ( %) for SH-MLs and NH-MLs, respectively. This is in line with the ozone loss mediated by VSLBr within the southern polar cap reported by Fernandez et al. (2017), who showed a maximum ozone depletion of up to −15 DU ( % of TOC) during October at the beginning of the century. Moreover, as mentioned above, the projected seasonal VSLBr impact on the TOC significantly decreases towards the end of the century, with springtime ΔTOC trends of up to 0.20 % and 0.14 % per decade for SH-MLs and NH-MLs, respectively (see Table 1). In comparison, the projected ΔTOC trends for summer and autumn reach approximately 0.10 % per decade at mid-latitudes. Note also that the changes in the magnitudes of these seasonal ΔTOC trends are mainly attributed to a marked seasonal difference of ozone loss mediated by VSLBr during the period of highest background halogen abundance (i.e. present day). These seasonal differences are reduced towards the end of the 21st century due to the decline in long-lived inorganic-halogen abundance.
Within the tropics, no significant changes in the TOC loss due to VSLBr are projected, though the model captures a slightly major TOC depletion during the 1990s. Therefore, VSLBr-driven ozone depletion is less sensitive to background halogen abundances, introducing an almost constant impact that is in line with its fixed emissions considered throughout the whole modelled period.
3.3 VSLBr impact on vertical ozone distributions
Timeline analysis of the annual mean stratospheric ozone difference between the experiments as a function of altitude at mid-latitudes and in the tropics is presented in Fig. 6. The inclusion of VSLBr produces a reduction in ozone concentrations (i.e. O3(z) number densities) throughout most of the stratosphere during the whole modelled period. The largest O3(z) reductions occur during the present-day period in the mid-latitude lowermost stratosphere, reaching ozone differences (ΔO3(z)) of up to −8 % ( molecules cm−3) and −5 % ( molecules cm−3) for SH-MLs and NH-MLs, respectively. Above 50 hPa (∼20 km), ΔO3(z) values are practically constant at around −1 % for all latitudes, which is linked to the lesser role played by VSL bromine compared to catalytic ozone loss by hydrogen () and nitrogen () oxides as well as by long-lived reactive halogens (Brasseur and Solomon, 2005; Salawitch et al., 2005; Müller, 2012).
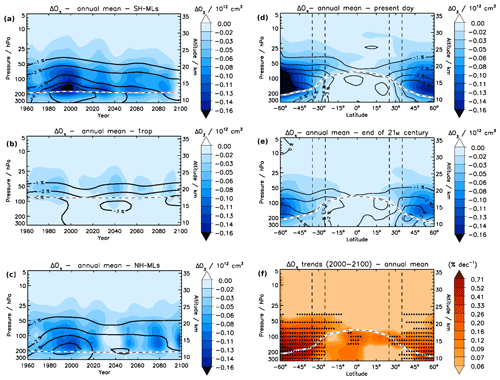
Figure 6Temporal evolution of the annual mean ozone difference (ΔO3) between experiments as a function of altitude (a) at SH-MLs, (b) in the tropics, and (c) at NH-MLs. The right panels show the zonal mean ΔO3 distributions for the (d) present day and (e) the end of the 21st century as well as the (f) ΔO3 trends (% per decade) over the century. The absolute (colour scale) and relative (contour line) ozone differences were calculated considering the ozone number densities (i.e. molecules cm−3) of each experiment. The masked regions in panel (f) indicate where the relative ΔO3(z) values between the present day and the end of the 21st century are statistically significant with a 95 % confidence interval using a two-tailed Student's t test.
Our modelled ΔO3(z) latitudinal distribution is in agreement with those reported by Sinnhuber and Meul et al. (2015) during the 1980–2005 period, with a larger percentage of ozone loss deepening around the mid-latitude tropopause. In addition, O3(z) reduction due to VSLBr in the mid-latitude lowermost stratosphere is about 50 % smaller by the end of the 21st century compared to the present-day period (see Fig. 6d and e). This is in line with projected local ΔO3(z) trends of up to 1 % and 0.5 % per decade at SH-MLs and NH-MLs, respectively (see Fig. 6f). The largest O3(z) reductions are located precisely at the same altitudes and latitudes where the ClOx∕Cly ratio peak is modelled within the lowermost stratosphere at SH-MLs (see Fig. 2), which explains the hemispheric asymmetry in the VSLBr impacts. This highlights that the enhancement in local ozone depletion mediated by VSLBr is largest in the context of maximum background active-chlorine abundance since they provide an additional partner for chlorine species involved in the ozone-depleting inter-halogen chemical reactions (e.g. ClOx–BrOx-Loss; see Sect. 3.4). These results are in agreement with Yang et al. (2014), who determined that VSLBr have a significantly larger ozone impact in the presence of a high stratospheric chlorine background than a low chlorine background using time slice sensitivity simulations with a variable and speculative VSLBr contribution on different background stratospheric Cly abundances.
Figure 7 shows the seasonal zonal mean distribution of the ΔO3(z) during the present-day period. The corresponding ΔO3(z) trends (% per decade) over the century are shown in Fig. S6. The largest contributions to the annual mean ozone loss in the mid-latitude lowermost stratosphere correspond to spring, followed by the preceding winter and the posterior summer in each hemisphere, with springtime ΔO3(z) reaching up to −10 % and −7 % for SH-MLs and NH-MLs, respectively (see Fig. 7b and d). This seasonal enhancement in VSLBr-mediated local ozone depletion is influenced by the seasonal changes in the heterogeneous chlorine reactivation processes on lowermost-stratospheric ice crystals since heterogeneous bromine reactivation occurs mainly in sulfate aerosols and therefore lacks seasonal changes (see Figs. S3 and S4). Consequently, since the seasonal changes in ozone loss are maximized during the present-day period, the largest local ΔO3(z) trends are also projected for spring and winter, with springtime ΔO3(z) trends reaching up to 1.6 % and 0.7 % per decade for SH-MLs and NH-MLs, respectively (see Fig. S6b and d).
Compared to the annual mean, springtime absolute O3(z) reductions along mid-latitudes are deepened over the first ∼5 km above the tropopause during the present-day period, whereas these reductions are located at higher altitudes (between 2 and 5 km above the tropopause) and over a narrower geographic area, mainly at NH-MLs, during summer and autumn. Therefore, VSL bromine introduces seasonal changes not only in overall O3(z) reduction but also in vertical O3(z) distribution. Moreover, since seasonal VSLBr impacts persist until the end of the century, they could lead to significantly different seasonal perturbations in the radiative effects mediated by ozone. Future studies are needed to explore the potential VSLBr-mediated seasonal effects on the atmosphere's radiative balance.
Near the tropical tropopause, the O3(z) reductions due to VSLBr are practically constant during the whole modelled period, with ΔO3(z) of approximately −2 % ( molecules cm−3) at 50 hPa (see Fig. 6b). Even with an increase in the contribution of to of up to 65 % by the end of century – due to the implementation of the Montreal Protocol (WMO, 2014, 2018) and the fixed stratospheric injection – ΔO3(z) changes in the tropical lowermost stratosphere are not significant over the century (see Fig. 6f). This is in line with the modelled impacts on the TOC. In contrast, at mid-latitudes, the seasonal VSLBr impacts on ozone significantly decrease over the century between 50 and 300 hPa (see Figs. 6f and S6a–d) even though the contribution percentage from to increases towards the end of the century. Moreover, the projected seasonal and annual ΔO3(z) trends peak precisely at the same altitudes where the modelled relative ΔO3(z) values are significant (see Figs. 6f and S6a–d). These results highlight the clear dependence of VSLBr impacts on the temporal evolution of background halogen abundances and the processes of heterogeneous halogen reactivation, which in turn depend on temperature as well as the formation and distribution of ice crystals and stratospheric sulfate aerosols. In fact, the projected impacts on vertical ozone distribution at the end of the century are similar to those prevailing before 1980 for both SH-MLs and NH-MLs since the background halogen abundances are similar between these periods. Thus, potential future changes in the lowermost stratospheric temperature (therefore influencing ice crystal formation), geoengineering of climate via injection of stratospheric sulfate, and the formation and distribution of stratospheric aerosol (e.g. future volcanic eruptions) will directly influence the processes of heterogeneous halogen reactivation and consequently VSLBr-driven ozone depletion efficiency (Tilmes et al., 2008, 2012; Banerjee et al., 2016; Klobas et al., 2017; Heckendorn et al., 2009).
3.4 Changes in the long-term seasonal evolution of halogen-driven ozone loss rate due to VSLBr
In order to determine the drivers controlling the VSLBr-driven seasonal impact on ozone in the lowermost stratosphere at mid-latitudes and in the tropics, we compute the odd-oxygen chemical loss from each ozone-depleting family considering the independent contributions of oxygen (Ox-Loss), hydrogen (HOx-Loss), nitrogen (NOx-Loss), and halogen (Halogx-Loss; Brasseur and Solomon, 2005; Saiz-Lopez et al., 2014). Moreover, we discriminate between the individual contributions of pure bromine (BrOx-Loss) and chlorine (ClOx-Loss) cycles and the inter-halogen (ClOx–BrOx-Loss) cycle to the halogen family (Halog). The odd-oxygen loss rate equations for the ozone-depleting families considered in this work are presented in Table S3.
Figure 8 shows the temporal evolution of the annual contribution percentage of each ozone-depleting family to the total ozone loss rate in the lowermost stratosphere at SH-MLs (∼120 hPa) for both experiments as well as the seasonal evolution of HOx-Loss and Halogx-Loss contributions in each experiment. The corresponding figures for the lowermost stratosphere at NH-MLs (∼120 hPa) and in the tropics (∼50 hPa) are shown in Figs. S7 and S8, respectively. Halogx-Loss represents the second most important contribution to the total ozone loss rate after HOx-Loss (∼80 %) at mid-latitudes in both experiments (see Figs. 8a and S7a), while within the tropics it represents the third family after HOx-Loss and NOx-Loss (see Fig. S8a). This partially explains the smaller modelled VSL bromine impact on ozone within the tropics as Halog represents at most 10 % of the total ozone destruction over this region. Moreover, the enhancement in the Halog contribution with respect to Halog by including VSLBr is mostly compensated by a decrease in the contribution at mid-latitudes. For example, for the present day, Halog shows an increase of ∼7 % with respect to Halog at SH-MLs, which is compensated by a reduction of ∼6 % in . In particular, the interaction between different catalytic ozone-depleting cycles involving OH and halogen radicals as well as the influence of reactive halogens in altering the OH∕HO2 ratio has been described elsewhere (Bloss et al., 2005; Saiz-Lopez and von Glasow, 2012).
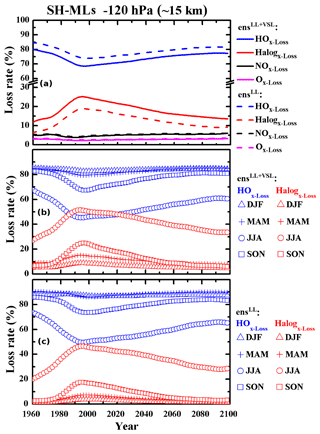
Figure 8Temporal evolution of the contribution percentages from different ozone-depleting families (Ox-Loss, NOx-Loss, HOx-Loss, Halogx-Loss) to the total odd-oxygen loss rate in the lowermost stratosphere (120 hPa) at SH-MLs. Panel (a) shows the annual mean contribution of each ozone-depleting family for the experiments with (solid line; ensLL+VSL) and without (dashed line; ensLL) VSLBr sources. Panels (b) and (c) show the seasonal mean contributions of both HOx-Loss and Halogx-Loss for the experiments with and without VSLBr sources, respectively.
Even though the additional seasonal ozone depletion due to VSLBr peaks during spring followed by winter at mid-latitudes, the largest Halogx-Loss contribution to the total ozone loss rate occurs during winter in both experiments, presenting a much smaller contribution during the rest of the year (see Figs. 8b, c and S7b, c). Indeed, this marked seasonal behaviour prevails both during boreal winter at NH-MLs and during the austral winter at SH-MLs. This is explained by considering the strong seasonal increase in active-chlorine abundance (driven by heterogeneous reactivation on ice crystals) as well as the winter decline in HOx abundance (driven by the decrease in solar radiation with respect to summertime). In fact, the enhancement in the Halog contribution due to VSLBr makes it the dominant ozone-depleting family during winter between 1985 and 2020 at SH-MLs, surpassing in importance the role played by the otherwise dominant HOx-Loss. In contrast, no seasonal variability of Halogx-Loss contribution is modelled within the tropics in either experiment (see Fig. S8b and c), and thus the seasonal VSLBr impact on ozone remains approximately constant during the whole modelled period around the annual mean.
Figure 9 shows the long-term evolution of the contribution percentage of BrOx-Loss, ClOx-Loss, and ClOx–BrOx-Loss to the Halogx-Loss as a function of months and years in the lowermost stratosphere at SH-MLs (120 hPa) for both experiments. Equivalent results are presented for NH-MLs and the tropics in Figs. S9 and S10, respectively. For any fixed year in both experiments, the BrOx-Loss contribution peaks during late summer and early autumn, while its minimum contribution occurs during the austral winter. In contrast, both the ClOx-Loss and ClOx–BrOx-Loss reach their maximum contribution during winter and early spring. Consequently, the inclusion of VSLBr increases the contribution in all seasons while also increasing the ClOx– contribution mainly in winter. This is at the expense of a decrease in the contribution. In addition, the wintertime ClOx– contribution reaches up to 50 % between 1990 and 2020, decreasing towards the end of the century as and abundances decrease within the A1 emission scenario. The decrease in both and ClOx– over time is offset by an increase in the contribution. Accordingly, the inclusion of VSLBr produces similar changes in the evolution of the contributions of each of the cycles that compose Halogx-Loss at NH-MLs, although the seasonal BrOx-Loss contribution is greater in both experiments compared to SH-MLs during the entire modelled period (see Fig. S9).
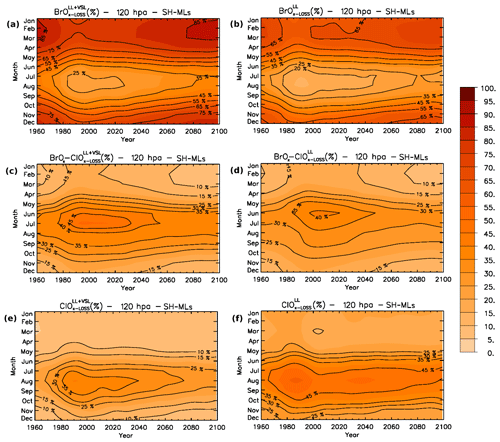
Figure 9Evolution of the (a, b) BrOx-Loss, (c, d) ClOx–BrOx-Loss, and (e, f) ClOx-Loss contribution percentages to Halogx-Loss as a function of the year and month in the lowermost stratosphere (120 hPa) at SH-MLs for the experiments (a, c, e) with and (b, d, f) without VSLBr sources.
In summary, the Halog contribution to the total ozone loss rate in summer and autumn is almost entirely dominated by . In the winter months, when the seasonal Halog contribution to the total ozone loss rate is comparatively higher, a transition from the contribution (∼50 %) at the beginning of the century towards at the end of the century is modelled. Thus, the halogen-driven ozone loss projected towards the end of the 21st century is mostly dominated by BrOx-Loss, leading to a less marked seasonal VSLBr impact on both vertical ozone distribution and the TOC, in accordance with the modelled results. On the other hand, within the tropics, dominates the seasonal Halog contribution for practically the entire modelled period (see Fig. S10), while represents a maximum contribution of up to ∼35 % during the end of the 20th century. The seasonal increase from to by including VSLBr is well marked and is practically offset by a reduction in the contribution. Furthermore, unlike mid-latitudes, ClOx– has a lower contribution to the tropical Halog, showing that VSLBr-driven topical ozone loss is independent of changes in the background halogen abundance throughout the modelled period.
This study has explored the impact of VSLBr on the temporal evolution of stratospheric ozone both in the late 20th century and in the course of the 21st century at mid-latitudes and in the tropics. The analysis compares two independent experiments, one of which considers only the anthropogenic LLCl and LLBr source contributions, while the other considers – in addition to long-lived sources – the contributions of VSLBr oceanic sources. We have evaluated annual and seasonal mean changes in vertical stratospheric ozone distribution and the TOC as well as examined the projected ozone depletion (ΔO3 and ΔTOC) trends over the century under a scenario in which both the relative contribution of VSLBr to total inorganic-bromine and the background inorganic-chlorine abundances shift towards the future. Finally, we have also assessed the long-term seasonal contribution changes of halogen-mediated ozone loss (Halogx-Loss) due to VSL bromine, focusing on the lowermost stratosphere and distinguishing the role of pure (BrOx-Loss, ClOx-Loss) and inter-halogen (ClOx–BrOx-Loss) cycles that compose Halogx-Loss. The results presented here highlight the importance of considering natural sources of halocarbons to determine the overall evolution of stratospheric ozone in the tropics and at mid-latitudes during the 21st century.
Our analysis shows that the inclusion of VSLBr results in a realistic stratospheric bromine loading that improves the agreement between the model and observations of the TOC at mid-latitudes, highlighting the need to consider these additional natural emissions to determine the overall evolution of ozone during the 21st century. Our results are in accordance with previous modelling studies that included natural bromocarbons and explored stratospheric ozone in the recent past (Salawitch et al., 2005; Feng et al., 2007; Sinnhuber et al., 2015) as well as by the end of the 21st century (Falk et al., 2017). However, unlike these previous works, we have explored seasonal ozone depletion arising from VSLBr with respect to the background stratospheric LLCl and LLBr abundances between 1960 and 2100.
At mid-latitudes, VSLBr introduce a continuous reduction in the TOC during the entire modelled period, with the largest TOC depletion occurring during the period of highest background halogen abundance (ΔTOC DU, −2.5 %, for SH-MLs and −5.5 DU, −1.6 %, for NH-MLs). In turn, a significant decrease in the impact towards the end of the 21st century at mid-latitudes is projected, with ΔTOC trends of 0.15 % and 0.10 % per decade for SH-MLs and NH-MLs, respectively. Even though the contribution of VSL bromine to total stratospheric bromine reaches almost 50 % by the end of the 21st century, our simulations show a statistically significant smaller VSLBr impact on lowermost stratospheric ozone as we move into the future. This result highlights that VSLBr-driven ozone loss is closely linked to the temporal evolution of LLCl and LLBr abundances regulated by the Montreal Protocol, as suggested by Yang et al. (2014). Along these same lines, Hossaini et al. (2015a) demonstrated that the chlorine load in the lowermost stratosphere has increased as a consequence of a recent and ongoing growth in emissions from anthropogenic VSLCl sources (mainly CH2Cl2) not controlled by the Montreal Protocol. If this VSLCl emissions trend continues into the future, additional studies will be required to quantify the increase in VSLBr impact modelled in this work on the projected ozone loss trends over the century considering the additional inorganic chlorine from VSLCl.
The largest TOC depletion associated with VSLBr corresponds to the spring months followed by winter, with maximum springtime ΔTOC2000 reaching −10 DU ( %) and −7.7 DU ( %) for SH-MLs and NH-MLs, respectively. We find that the inclusion of VSLBr leads to seasonal changes in both local O3(z) reduction and its vertical ΔO3(z) distribution within the lowermost stratosphere, which could result in different seasonal perturbations in the radiative effects mediated by ozone. This seasonal enhancement in VSLBr-mediated local ozone loss is strongly influenced by the seasonal changes in the processes of heterogeneous chlorine activation on ice crystals (mainly by ClONO2 and HCl recycling). In fact, the modelled additional increase in heterogeneous chlorine reactivation efficiency in the lowermost stratosphere at SH-MLs compared to NH-MLs leads to hemispheric asymmetry in the VSLBr-driven ozone reductions.
Even though the additional seasonal ozone depletion due to VSLBr peaks during spring followed by winter, the largest Halog contribution to the total ozone loss rate occurs during winter, with a much smaller contribution during all other seasons. In fact, the wintertime Halog contribution dominates the total ozone loss rate between 1985 and 2020 in the lowermost stratosphere at SH-MLs, with a ClOx– contribution to Halog reaching up to 50%. Due to the expected decrease in the LLCl and LLBr abundances, a transition from ClOx– to BrOx-Loss during the course of the 21st century is projected, leading to a less marked seasonal VSLBr impact towards the end of the century.
Within the tropics, although our model captures a slightly greater TOC depletion associated with VSLBr during the 1990s, no significant change is projected by the end of the 21st century. Halogx-Loss represents the third family in the contribution to the total ozone loss rate after HOx-Loss and NOx-Loss in the tropical lowermost stratosphere, with an almost constant seasonal contribution throughout the modelled period. practically dominates the contribution to Halog, with a maximum ClOx– contribution of up to ∼30 % by the end of the 20th century, reflecting the low sensitivity of VSL bromine to background halogen abundances to drive stratospheric ozone depletion.
Computing resources, support, and data storage are provided and maintained by the Computational and Information System Laboratory from the National Center of Atmospheric Research (CISL, 2017). The code of the CAM-chem model can be downloaded from the website (https://www2.acom.ucar.edu/gcm/cam-chem (last access: 10 March 2018) (Atmospheric Chemistry Observations and Modelling Division repository, 2018). Data that support the findings of this study can be downloaded from the AC2 web page (https://ac2.iqfr.csic.es/en/publications, last access: 25 June 2020) (Atmospheric Chemistry and Climate Department, 2020).
The supplement related to this article is available online at: https://doi.org/10.5194/acp-20-8083-2020-supplement.
RPF and ASL designed the experiments. RPF and JAB configured and ran all the simulations. JAB processed the results of all simulations, led the manuscript, and prepared the figures. RPF, ASL, FIS, and CAC provided additional feedback on geophysical processing and representation. JAB, RPF, FIS, CAC, JFL, and ASL were involved in the discussion and iterations of the manuscript.
The authors declare that they have no conflict of interest.
Javier Alejandro Barrera and Rafael Pedro Fernandez would like to thank CONICET, UNCuyo (SeCTyP M032/3853), and UTN (PID 4920-194/2018) for additional financial support. Alfonso Saiz-Lopez, Carlos Alberto Cuevas, and Fernando Iglesias-Suarez are supported by the Consejo Superior de Investigaciones Científicas (CSIC) of Spain. We are very thankful to Douglas Kinnison for very helpful discussions and suggestions. We thank the two anonymous reviewers for their helpful comments that improved this paper.
This research has been supported by the European Research Council Executive Agency under the European Union's Horizon 2020 Research and Innovation programme (grant no. ERC-2016-COG 726349 CLIMAHAL) and the Agencia Nacional de Promoción Científica y Técnica (grant no. ANPCYT PICT-2016-0714).
This paper was edited by Marc von Hobe and reviewed by two anonymous referees.
Abrahamsson, K., Granfors, A., Ahnoff, M., Cuevas, C. A., and Saiz-Lopez, A.: Organic bromine compounds produced in sea ice in Antarctic winter, Nat. Commun., 9, 5291–5302, https://doi.org/10.1038/s41467-018-07062-8, 2018.
Anderson, J. G. and Clapp, C. E.: Coupling free radical catalysis, climate change, and human health, Phys. Chem. Chem. Phys., 20, 10569–10587, https://doi.org/10.1039/C7CP08331A, 2018.
Anderson, J. G., Wilmouth, D. M., Smith, J. B., and Sayres, D. S.: UV Dosage Levels in Summer: Increased Risk of Ozone Loss from Convectively Injected Water Vapor, Science, 337, 835–839, https://doi.org/10.1126/science.1222978, 2012.
Anderson, J. G., Weisenstein, D. K., Bowman, K. P., Homeyer, C. R., Smith, J. B., Wilmouth, D. M., Sayres, D. S., Klobas, J. E., Leroy, S. S., Dykema, J. A., and Wofsy, S. C.: Stratospheric ozone over the United States in summer linked to observations of convection and temperature via chlorine and bromine catalysis, P. Natl. Acad. Sci. USA, 114, 4905–4913, https://doi.org/10.1073/pnas.1619318114, 2017.
Atmospheric Chemistry and Climate Department: CSIC, available at: https://ac2.iqfr.csic.es/en/publications, last access: 25 June 2020.
Atmospheric Chemistry Observations and Modelling Division repository: NCAR, available at: https://www2.acom.ucar.edu/gcm/cam-chem, last access: 10 March 2018.
Banerjee, A., Maycock, A. C., Archibald, A. T., Abraham, N. L., Telford, P., Braesicke, P., and Pyle, J. A.: Drivers of changes in stratospheric and tropospheric ozone between year 2000 and 2100, Atmos. Chem. Phys., 16, 2727–2746, https://doi.org/10.5194/acp-16-2727-2016, 2016.
Bhartia, P. K., McPeters, R. D., Flynn, L. E., Taylor, S., Kramarova, N. A., Frith, S., Fisher, B., and DeLand, M.: Solar Backscatter UV (SBUV) total ozone and profile algorithm, Atmos. Meas. Tech., 6, 2533–2548, https://doi.org/10.5194/amt-6-2533-2013, 2013.
Bloss, W. J., Lee, J. D., Johnson, G. P., Sommariva, R., Heard, D. E., Saiz-Lopez, A., Plane, J. M. C., McFiggans, G., Coe, H., Flynn, M., Williams, P., Rickard, A. R., and Fleming, Z. L.: Impact of halogen monoxide chemistry upon boundary layer OH and HO2 concentrations at a coastal site, Geophys. Res. Lett., 32, L06814, https://doi.org/10.1029/2004GL022084, 2005.
Brasseur, G. and Solomon, S.: Aeronomy of the Middle Atmosphere: Chemistry and Physics of the Stratosphere and Mesosphere, in: chap. 5, 3rd Edn., Springer, Dordrecht, the Netherlands, 265–442, 2005.
Bregman, B., Wang, P. H., and Lelieveld, J.: Chemical ozone loss in the tropopause region on subvisible ice clouds, calculated with a chemistry-transport model, J. Geophys. Res., 107, 4032, https://doi.org/10.1029/2001JD000761, 2002.
Borrmann, S., Solomon, S., Dye, J. E., and Luo, B. P.: The potential of cirrus clouds for heterogeneous chlorine activation, Geophys. Res. Lett., 23, 2133–2136, https://doi.org/10.1029/96GL01957, 1996.
Borrmann, S., Solomon, S., Avallone, L., Toohey, D., and Baumgardner, D.: On the occurrence of ClO in cirrus clouds and volcanic aerosol in the tropopause region, Geophys. Res. Lett., 24, 2011–2014, https://doi.org/10.1029/97GL02053, 1997.
Carpenter, L. J. and Liss, P. S.: On temperate sources of bromoform and other reactive organic bromine gases, J. Geophy. Res., 105, 20539–20547, https://doi.org/10.1029/2000JD900242, 2000.
Carpenter, L. J., Wevill, D. J., Hopkins, J. R., Dunk, R. M., Jones, C. E., Hornsby, K. E., and McQuaid, J. B.: Bromoform in tropical Atlantic air from 25∘ N to 25∘ S, Geophys. Res. Lett., 34, L11810, https://doi.org/10.1029/2007GL029893, 2007.
Chipperfield, M. P., Bekki, S., Dhomse, S., Harris, N. R. P., Hassler, B., Hossaini, R., Steinbrecht, W., Thieblemont, R., and Weber, M.: Detecting recovery of the stratospheric ozone layer, Nature, 549, 211–218, https://doi.org/10.1038/nature23681, 2017.
CISL – Computational and Information Systems Laboratory: Cheyenne: HPE/SGI ICE XA System (NCAR Community Computing), National Center for Atmospheric Reserach (NCAR), Boulder, CO, USA, https://doi.org/10.5065/D6RX99HX, 2017.
Daniel, J. S., Solomon, S., Portmann, R. W., and Garcia, R. R.: Stratospheric ozone destruction: The importance of bromine relative to chlorine, J. Geophys. Res., 104, 23871, https://doi.org/10.1029/1999JD900381, 1999.
DeLand, M. T., Taylor, S. L., Huang, L. K., and Fisher, B. L.: Calibration of the SBUV version 8.6 ozone data product, Atmos. Meas. Tech., 5, 2951–2967, https://doi.org/10.5194/amt-5-2951-2012, 2012.
Dessler, A. E.: Clouds and water vapor in the Northern Hemisphere summertime stratosphere, J. Geophys. Res., 114, D00H09, https://doi.org/10.1029/2009JD012075, 2009.
Dhomse, S. S., Kinnison, D., Chipperfield, M. P., Salawitch, R. J., Cionni, I., Hegglin, M. I., Abraham, N. L., Akiyoshi, H., Archibald, A. T., Bednarz, E. M.., Bekki, S., Braesicke, P., Butchart, N., Dameris, M., Deushi, M., Frith, S., Hardiman, S. C., Hassler, B., Horowitz, L. W., Hu, R.-M., Jöckel, P., Josse, B., Kirner, O., Kremser, S., Langematz, U., Lewis, J., Marchand, M., Lin, M., Mancini, E., Marécal, V., Michou, M., Morgenstern, O., O'Connor, F. M., Oman, L., Pitari, G., Plummer, D. A., Pyle, J. A., Revell, L. E., Rozanov, E., Schofield, R., Stenke, A., Stone, K., Sudo, K., Tilmes, S., Visioni, D., Yamashita, Y., y Zeng, G.: Estimates of ozone return dates from Chemistry-Climate Model Initiative simulations, Atmos. Chem. Phys., 18, 8409–8438, https://doi.org/10.5194/acp-18-8409-2018, 2018.
Dorf, M., Bösch, H., Butz, A., Camy-Peyret, C., Chipperfield, M. P., Engel, A., Goutail, F., Grunow, K., Hendrick, F., Hrechanyy, S., Naujokat, B., Pommereau, J.-P., Van Roozendael, M., Sioris, C., Stroh, F., Weidner, F., and Pfeilsticker, K.: Balloon-borne stratospheric BrO measurements: comparison with Envisat/SCIAMACHY BrO limb profiles, Atmos. Chem. Phys., 6, 2483–2501, https://doi.org/10.5194/acp-6-2483-2006, 2006.
Drdla, K. and Müller, R.: Temperature thresholds for chlorine activation and ozone loss in the polar stratosphere, Ann. Geophys., 30, 1055–1073, https://doi.org/10.5194/angeo-30-1055-2012, 2012.
Dvortsov, V. L., Geller, M. A., Solomon, S., Schauffler, S. M., Atlas, E. L., Blake, D. R.: Rethinking reactive halogen budgets in the midlatitude lower stratosphere, Geophys. Res. Lett., 26, 1699–1702, https://doi.org/10.1029/1999GL900309, 1999.
Eyring, V., Cionni, I., Bodeker, G. E., Charlton-Perez, A. J., Kinnison, D. E., Scinocca, J. F., Waugh, D. W., Akiyoshi, H., Bekki, S., Chipperfield, M. P., Dameris, M., Dhomse, S., Frith, S. M., Garny, H., Gettelman, A., Kubin, A., Langematz, U., Mancini, E., Marchand, M., Nakamura, T., Oman, L. D., Pawson, S., Pitari, G., Plummer, D. A., Rozanov, E., Shepherd, T. G., Shibata, K., Tian, W., Braesicke, P., Hardiman, S. C., Lamarque, J. F., Morgenstern, O., Pyle, J. A., Smale, D., and Yamashita, Y.: Multi-model assessment of stratospheric ozone return dates and ozone recovery in CCMVal-2 models, Atmos. Chem. Phys., 10, 9451–9472, https://doi.org/10.5194/acp-10-9451-2010, 2010.
Eyring, V., Lamarque, J.-F., Hess, P., Arfeuille, F., Bowman, K., Chipperfield, M. P., Duncan, B., Fiore, A., Gettelman, A., Giorgetta, M. A., Granier, C., Hegglin, M., Kinnison, D., Kunze, M., Langematz, U., Luo, B., Martin, R., Matthes, K., Newman, P. A., Peter, T., Robock, A., Ryerson, T., Saiz-Lopez, A., Salawitch, R., Schultz, M., Shepherd, T. G., Shindell, D., Stähelin, J., Tegtmeier, S., Thomason, L., Tilmes, S., Vernier, J.-P., Waugh, D. W. and Young, P. J.: Overview of IGAC/SPARC Chemistry-Climate Model Initiative (CCMI) Community Simulations in Support of Upcoming Ozone and Climate Assessments, SPARC Newslett., 40, 48–66, 2013.
Falk, S., Sinnhuber, B.-M., Krysztofiak, G., Jöckel, P., Graf, P., and Lennartz, S. T.: Brominated VSLS and their influence on ozone under a changing climate, Atmos. Chem. Phys., 17, 11313–11329, https://doi.org/10.5194/acp-17-11313-2017, 2017.
Feng, W., Chipperfield, M. P., Dorf, M., Pfeilsticker, K., and Ricaud, P.: Mid-latitude ozone changes: studies with a 3-D CTM forced by ERA-40 analyses, Atmos. Chem. Phys., 7, 2357–2369, https://doi.org/10.5194/acp-7-2357-2007, 2007.
Fernandez, R. P., Salawitch, R. J., Kinnison, D. E., Lamarque, J.-F. and Saiz-Lopez, A.: Bromine partitioning in the tropical tropopause layer: implications for stratospheric injection, Atmos. Chem. Phys., 14, 13391–13410, https://doi.org/10.5194/acp-14-13391-2014, 2014.
Fernandez, R. P., Kinnison, D. E., Lamarque, J.-F., Tilmes, S., and Saiz-Lopez, A.: Impact of biogenic very short-lived bromine on the Antarctic ozone hole during the 21st century, Atmos. Chem. Phys., 17, 1673–1688, https://doi.org/10.5194/acp-17-1673-2017, 2017.
Frith, S. M., Kramarova, N. A., Stolarski, R. S., McPeters, R. D., Bhartia, P. K., and Labow, G. J.: Recent changes in total column ozone based on the SBUV Version 8.6 Merged Ozone Data Set, J. Geophys. Res.-Atmos., 119, 9735–9751, https://doi.org/10.1002/2014JD021889, 2014.
Frith, S. M., Stolarski, R. S., Kramarova, N. A., and McPeters, R. D.: Estimating uncertainties in the SBUV Version 8.6 merged profile ozone data set, Atmos. Chem. Phys., 17, 14695–14707, https://doi.org/10.5194/acp-17-14695-2017, 2017.
Heckendorn, P., Weisenstein, D., Fueglistaler, S., Luo, B., Rozanov, E., Schraner, M., Thomason, L., and Peter, T.: The impact of geo-engineering aerosols on stratospheric temperature and ozone, Environ. Res. Lett., 4, 045108, https://doi.org/10.1088/1748-9326/4/4/045108, 2009.
Hendrick, F., Van Roozendael, M., Chipperfield, M. P., Dorf, M., Goutail, F., Yang, X., Fayt, C., Hermans, C., Pfeilsticker, K., Pommereau, J.-P., Pyle, J. A., Theys, N., and De Maziẽre, M.: Retrieval of stratospheric and tropospheric BrO profiles and columns using ground-based zenith-sky DOAS observations at Harestua, 60∘ N, Atmos. Chem. Phys., 7, 4869–4885, https://doi.org/10.5194/acp-7-4869-2007, 2007.
Hendrick, F., Johnston, P. V., Kreher, K., Hermans, C., De Maziere, M., and Van Roozendael, M.: One decade trend analysis of stratospheric BrO over Harestua (60∘ N) and Lauder (44∘ S) reveals a decline, Geophys. Res. Lett., 35, L14801, https://doi.org/10.1029/2008GL034154, 2008.
Hossaini, R., Chipperfield, M. P., Saiz-Lopez, A., Harrison, J. J., von Glasow, R., Sommariva, R., Atlas, E., Navarro, M., Montzka, S. A., Feng, W., Dhomse, S., Harth, C., Mühle, J., Lunder, C., O'Doherty, S., Young, D., Reimann, S., Vollmer, M. K., Krummel, P. B., and Bernath, P. F.: Growth in stratospheric chlorine from short-lived chemicals not controlled by the Montreal Protocol, Geophys. Res. Lett., 42, 4573–4580, https://doi.org/10.1002/2015GL063783, 2015a.
Hossaini, R., Chipperfield, M. P., Montzka, S. A., Rap, A., Dhomse, S., and Feng, W.: Efficiency of short-lived halogens at influencing climate through depletion of stratospheric ozone, Nat. Geosci., 8, 186–190, https://doi.org/10.1038/NGEO2363, 2015b.
Hossaini, R., Chipperfield, M. P., Montzka, S. A., Leeson, A. A., Dhomse, S., and Pyle, J. A.: The increasing threat to stratospheric ozone from dichloromethane, Nat. Commun., 8, 15962, https://doi.org/10.1038/ncomms15962, 2017.
Iglesias-Suarez, F., Kinnison, D. E., Rap, A., Maycock, A. C., Wild, O., and Young, P. J.: Key drivers of ozone change and its radiative forcing over the 21st century, Atmos. Chem. Phys., 18, 6121–6139, https://doi.org/10.5194/acp-18-6121-2018, 2018.
Klobas, J. E., Wilmouth, D. M., Weisenstein, D. K., Anderson, J. G., and Salawitch, R. J.: Ozone depletion following future volcanic eruptions, Geophys. Res. Lett., 44, 7490–7499, https://doi.org/10.1002/2017GL073972, 2017.
Lamarque, J.-F., Kinnison, D. E., Hess, P. G., and Vitt, F. M.: Simulated lower stratospheric trends between 1970 and 2005: Identifying the role of climate and composition changes, J. Geophys. Res., 113, D12301, https://doi.org/10.1029/2007JD009277, 2008.
Lamarque, J.-F., Emmons, L. K., Hess, P. G., Kinnison, D. E., Tilmes, S., Vitt, F., Heald, C. L., Holland, E. A., Lauritzen, P. H., Neu, J., Orlando, J. J., Rasch, P. J., and Tyndall, G. K.: CAM-chem: description and evaluation of interactive atmospheric chemistry in the Community Earth System Model, Geosci. Model Dev., 5, 369–411, https://doi.org/10.5194/gmd-5-369-2012, 2012.
Leedham Elvidge, E. C., Oram, D. E., Laube, J. C., Baker, A. K., Montzka, S. A., Humphrey, S., O'Sullivan, D. A., and Brenninkmeijer, C. A. M.: Increasing concentrations of dichloromethane, CH2Cl2, inferred from CARIBIC air sam ples collected 1998–2012, Atmos. Chem. Phys., 15, 1939–1958, https://doi.org/10.5194/acp-15-1939-2015, 2015.
Lennartz, S. T., Krysztofiak, G., Marandino, C. A., Sinnhuber, B.-M., Tegtmeier, S., Ziska, F., Hossaini, R., Krüger, K., Montzka, S. A., Atlas, E., Oram, D. E., Keber, T., Bönisch, H., and Quack, B.: Modelling marine emissions and atmospheric distributions of halocarbons and dimethyl sulfide: the influence of prescribed water concentration vs. prescribed emissions, Atmos. Chem. Phys., 15, 11753–11772, https://doi.org/10.5194/acp-15-11753-2015, 2015.
Livesey, N. J., Read, W. G., Wagner, P. A., Froidevaux, L., Lambert, A., Manney, G. L., F., L., Valle, M., Pumphrey, H. C., Santee, M. L., Schwartz, M. J., Wang, S., Fuller, R. A., Jarnot, R. F., Knosp, B. W., Martinez, E., and Lay, R. R.: Version 4.2x Level 2 data quality and description document, JPL D-33509, Rev. D., Jet Propulsion Lab, available at: https://mls.jpl.nasa.gov/data/v4-2_data_quality_document.pdf (last access: 20 July 2019), 2018.
Marsh, D. R., Mills, M. J., Kinnison, D. E., Lamarque, J.-F., Calvo, N., and Polvani, L. M.: Climate Change from 1850 to 2005 Simulated in CESM1(WACCM), J. Climate, 26, 7372–7391, https://doi.org/10.1175/JCLI-D-12-00558.1, 2013.
McElroy, M. B., Salawitch, R. J., Wofsy, S. C., and Logan, J. A.: Reductions of Antarctic ozone due to synergistic interactions of chlorine and bromine, Nature, 321, 759–762, 1986.
Meinshausen, M., Smith, S. J., Calvin, K., Daniel, J. S., Kainuma, M. L. T., Lamarque, J. F., Matsumoto, K., Montzka, S. A., Raper, S. C. B., Riahi, K., Thomson, A., Velders, G. J. M., and Vuuren, D. P. P.: The RCP greenhouse gas concentrations and their extensions from 1765 to 2300, Climatic Change, 109, 213–241, https://doi.org/10.1007/s10584-011-0156-z, 2011.
Müller, R. (Ed.): Stratospheric Ozone Depletion and Climate Change, in: chap 3, RSC Publishing, Cambridge, 78–99, 2012.
Neale, R. B., Richter, J., Park, S., Lauritzen, P. H., Vavrus, S. J., Rasch, P. J., and Zhang, M.: The Mean Climate of the Community Atmosphere Model (CAM4) in Forced SST and Fully Coupled Experiments, J. Climate, 26, 5150–5168, https://doi.org/10.1175/JCLI-D-12-00236.1, 2013.
Ordóñez, C., Lamarque, J.-F., Tilmes, S., Kinnison, D. E., Atlas, E. L., Blake, D. R., Sousa Santos, G., Brasseur, G. and Saiz-Lopez, A.: Bromine and iodine chemistry in a global chemistry-climate model: description and evaluation of very short-lived oceanic sources, Atmos. Chem. Phys., 12, 1423–1447, https://doi.org/10.5194/acp-12-1423-2012, 2012.
Prather, M. J. and Watson, R. T.: Stratospheric ozone depletion and future levels of atmospheric chlorine and bromine, Nature, 344, 729–734, 1990.
Quack, B. and Wallace, D. W. R.: Air-sea flux of bromoform: Controls, rates, and implications, Global Biochem. Cy., 17, 1023, https://doi.org/10.1029/2002GB001890, 2003.
Quack, B., Peeken, I., Petrick, G., and Nachtigall, K.: Oceanic distribution and sources of bromoform and dibromomethane in the Mauritanian upwelling, J. Geophys. Res., 112, C10006, https://doi.org/10.1029/2006JC003803, 2007.
Riese, M., Ploeger, F., Rap, A., Vogel, B., Konopka, P., Dameris, M., and Forster, P.: Impact of uncertainties in atmospheric mixing on simulated UTLS composition and related radiative effects, J. Geophys. Res.-Atmos., 117, D16305, https://doi.org/10.1029/2012jd017751, 2012.
Robrecht, S., Vogel, B., Grooß, J.-U., Rosenlof, K., Thornberry, T., Rollins, A., Krämer, M., Christensen, L., and Müller, R.: Mechanism of ozone loss under enhanced water vapour conditions in the mid-latitude lower stratosphere in summer, Atmos. Chem. Phys., 19, 5805–5833, https://doi.org/10.5194/acp-19-5805-2019, 2019.
Rolf, C., Afchine, A., Bozem, H., Buchholz, B., Ebert, V., Guggenmoser, T., Hoor, P., Konopka, P., Kretschmer, E., Müller, S., Schlager, H., Spelten, N., Sumińska-Ebersoldt, O., Ungermann, J., Zahn, A., and Krämer, M.: Transport of Antarctic stratospheric strongly dehydrated air into the troposphere observed during the HALO-ESMVal campaign 2012, Atmos. Chem. Phys., 15, 9143–9158, https://doi.org/10.5194/acp-15-9143-2015, 2015.
Saiz-Lopez, A. and von Glasow, R.: Reactive halogen chemistry in the troposphere, Chem. Soc. Rev., 41, 6448–6472, https://doi.org/10.1039/c2cs35208g, 2012.
Saiz-Lopez, A., Fernandez, R. P., Ordóñez, C., Kinnison, D. E., Gómez Martín, J. C., Lamarque, J.-F., and Tilmes, S.: Iodine chemistry in the troposphere and its effect on ozone, Atmos. Chem. Phys., 14, 13119–13143, https://doi.org/10.5194/acp-14-13119-2014, 2014.
Salawitch, R. J., Weisenstein, D. K., Kovalenko, L. J., Sioris, C. E., Wennberg, P. O., Chance, K., Ko, M. K. W., and McLinden, C. A.: Sensitivity of ozone to bromine in the lower stratosphere, Geophys. Res. Lett., 32, L05811, https://doi.org/10.1029/2004GL021504, 2005.
Salawitch, R. J., Canty, T., Kurosu, T., Chance, K., Liang, Q., da Silva, A., Pawson, S., Nielsen, J. E., Rodriguez, J. M., Bhartia, P. K., Liu, X., Huey, L. G., Liao, J., Stickel, R. E., Tanner, D. J., Dibb, J. E., Simpson, W. R., Donohoue, D., Weinheimer, A., Flocke, F., Knapp, D., Montzka, D., Neuman, J. A., Nowak, J. B., Ryerson, T. B., Oltmans, S., Blake, D. R., Atlas, E. L., Kinnison, D. E., Tilmes, S., Pan, L. L., Hendrick, F., Van Roozendael, M., Kreher, K., Johnston, P. V., Gao, R. S., Johnson, B., Bui, T. P., Chen, G., Pierce, R. B., Crawford, J. H., and Jacob, D. J.: A new interpretation of total column BrO during Arctic spring, Geophys. Res. Lett., 37, L21805, https://doi.org/10.1029/2010GL043798, 2010.
Santee, M. L., Manney, G. L., Livesey, N. J., Froidevaux, L., Schwartz, M. J., and Read, W. G.: Trace gas evolution in the lowermost stratosphere from Aura Microwave Limb Sounder measurements, J. Geophys. Res., 116, D18306, https://doi.org/10.1029/2011JD015590, 2011.
Sinnhuber, B.-M., Sheode, N., Sinnhuber, M., Chipperfield, M. P., and Feng, W.: The contribution of anthropogenic bromine emissions to past stratospheric ozone trends: a modelling study, Atmos. Chem. Phys., 9, 2863–2871, https://doi.org/10.5194/acp-9-2863-2009, 2009.
Sinnhuber, B.-M. and Meul, S.: Simulating the impact of emissions of brominated very short lived substances on past stratospheric ozone trends, Geophys. Res. Lett., 2449–2456, https://doi.org/10.1002/2014GL062975, 2015.
Solomon, S.: Stratospheric ozone depletion: A review of concepts and history, Rev. Geosphys., 37, 275–316, https://doi.org/10.1029/1999RG900008, 1999.
Solomon, S., Borrmann, S., Garcia, R. R., Portmann, R., Thomason, L., Poole, L. R., Winker, D., and McCormick, M. P.: Heterogeneous chlorine chemistry in the tropopause region, J. Geophys. Res., 102, 21411–21429, https://doi.org/10.1029/97JD01525, 1997.
Solomon, S., Ivy, D. J., Kinnison, D., Mills, M. J., Neely, R. R., and Schmidt, A.: Emergence of healing in the Antarctic ozone layer, Science, 353, 269–274, https://doi.org/10.1126/science.aae0061, 2016a.
Solomon, S., Kinnison, D., Garcia, R. R., Bandoro, J., Mills, M., Wilka, C., Neely III, R. R., Schmidt, A., Barnes, J. E., Vernier, J., and Höpfner, M.: Monsoon circulations and tropical heterogeneous chlorine chemistry in the stratosphere, Geophys. Res. Lett., 43, 12624–12633, https://doi.org/10.1002/2016GL071778, 2016b.
Spang, R., Günther, G., Riese, M., Hoffmann, L., Müller, R., and Griessbach, S.: Satellite observations of cirrus clouds in the Northern Hemisphere lowermost stratosphere, Atmos. Chem. Phys., 15, 927–950, https://doi.org/10.5194/acp-15-927-2015, 2015.
Strahan, S. E. and Douglass, A. R.: Decline in Antarctic Ozone Depletion and Lower Stratospheric Chlorine Determined From Aura Microwave Limb Sounder Observations, Geophys. Res. Lett., 45, 382–390, https://doi.org/10.1002/2017GL074830, 2018.
Tilmes, S., Müller, R., and Salawitch, R.: The sensitivity of polar ozone depletion to proposed geoengineering schemes, Science, 320, 1201–1204, https://doi.org/10.1126/science.1153966, 2008.
Tilmes, S., Kinnison, D. E., Garcia, R. R., Salawitch, R., Canty, T., Lee-Taylor, J., Madronich, S., and Chance, K.: Impact of very short-lived halogens on stratospheric ozone abundance and UV radiation in a geo-engineered atmosphere, Atmos. Chem. Phys., 12, 10945–10955, https://doi.org/10.5194/acp-12-10945-2012, 2012.
Tilmes, S., Lamarque, J., Emmons, L. K., Kinnison, D. E., Marsh, D., Garcia, R. R., Smith, A. K., Neely, R. R., Conley, A., Vitt, F., Martin, M. V., Tanimoto, H., Simpson, I., Blake, D. R. and Blake, N.: Representation of the Community Earth System Model (CESM1) CAM4-chem within the Chemistry-Climate Model Initiative (CCMI), Geosci. Model Dev., 9, 1853–1890, https://doi.org/10.5194/gmd-9-1853-2016, 2016.
Thornton, B. F., Toohey, D. W., Avallone, L. M., Harder, H., Martinez, M., Simpas, J. B., Brune, W. H., and Avery, M. A.: In situ observations of ClO near the winter polar tropopause, J. Geophys. Res., 108, 8333, https://doi.org/10.1029/2002JD002839, 2003.
von Hobe, M., Grooß, J.-U., Günther, G., Konopka, P., Gensch, I., Krämer, M., Spelten, N., Afchine, A., Schiller, C., Ulanovsky, A., Sitnikov, N., Shur, G., Yushkov, V., Ravegnani, F., Cairo, F., Roiger, A., Voigt, C., Schlager, H., Weigel, R., Frey, W., Borrmann, S., Müller, R., and Stroh, F.: Evidence for heterogeneous chlorine activation in the tropical UTLS, Atmos. Chem. Phys., 11, 241–256, https://doi.org/10.5194/acp-11-241-2011, 2011.
Warwick, N. J., Pyle, J. A., Carver, G. D., Yang, X., Savage, N. H., O'Connor, F. M., and Cox, R. A.: Global modeling of biogenic bromocarbons, J. Geophys. Res., 111, D24305, https://doi.org/10.1029/2006JD007264, 2006.
Waters, J. W., Froidevaux, L., Harwood, R. S., Jarnot, R. F., Pickett, H. M., Read, W. G., Siegel, P. H., Cofield, R. E., Filipiak, M. J., Flower, D. A., Holden, J. R., Lau, G. K., Livesey, N. J., Manney, G. L., Pumphrey, H. C., Santee, M. L., Wu, D. L., Cuddy, D. T., Lay, R. R., Loo, M. S., Perun, V. S., Schwartz, M. J., Stek, P. C., Thurstans, R. P., Boyles, M. A., Chandra, K. M., Chavez, M. C., Chen, G. S., Chudasama, B. V., Dodge, R., Fuller, R. A., Girard, M. A., Jiang, J. H., Jiang, Y., Knosp, B. W., Labelle, R. C., Lam, J. C., Lee, K. A., Miller, D., Oswald, J. E., Patel, N. C., Pukala, D. M., Quintero, O., Scaff, D. M., Van Snyder, W., Tope, M. C., Wagner, P. A., and Walch, M. J.: The Earth Observing System Microwave Limb Sounder (EOS MLS) on the Aura satellite, IEEE T. Geosci. Remote, 44, 1075–1092, https://doi.org/10.1109/TGRS.2006.873771, 2006.
Weber, M., Coldewey-Egbers, M., Fioletov, V. E., Frith, S. M., Wild, J. D., Burrows, J. P., Long, C. S., and Loyola, D.: Total ozone trends from 1979 to 2016 derived from five merged observational datasets – the emergence into ozone recovery, Atmos. Chem. Phys., 18, 2097–2117, https://doi.org/10.5194/acp-18-2097-2018, 2018.
WMO – World Meteorological Organization: Scientific Assessment of Ozone Depletion: 2010, Global Ozone Research and Monitoring Project-Report No. 52, Geneva, Switzerland, 2011.
WMO – World Meteorological Organization: Scientific Assessment of Ozone Depletion: 2014, Global Ozone Research and Monitoring Project-Report No. 55, Geneva, Switzerland, 2014.
WMO – World Meteorological Organization: Scientific Assessment of Ozone Depletion: 2018, Global Ozone Research and Monitoring Project-Report No. 58, Geneva, Switzerland, 2018.
Wofsy, S. C., McElroy, M. B., and Yung, Y. L.: The chemistry of atmospheric bromine, Geophys. Res. Lett., 2, 215–218, https://doi.org/10.1029/GL002i006p00215, 1975.
Yang, X., Abraham, N. L., Archibald, A. T., Braesicke, P., Keeble, J., Telford, P. J., Warwick, N. J., and Pyle, J. A.: How sensitive is the recovery of stratospheric ozone to changes in concentrations of very short-lived bromocarbons?, Atmos. Chem. Phys., 14, 10431–10438, https://doi.org/10.5194/acp-14-10431-2014, 2014.
Ziska, F., Quack, B., Tegtmeier, S., Stemmler, I., and Krüger, K.: Future emissions of marine halogenated very-short lived substances under climate change, J. Atmos. Chem., 74, 245–260, https://doi.org/10.1007/s10874-016-9355-3, 2017.